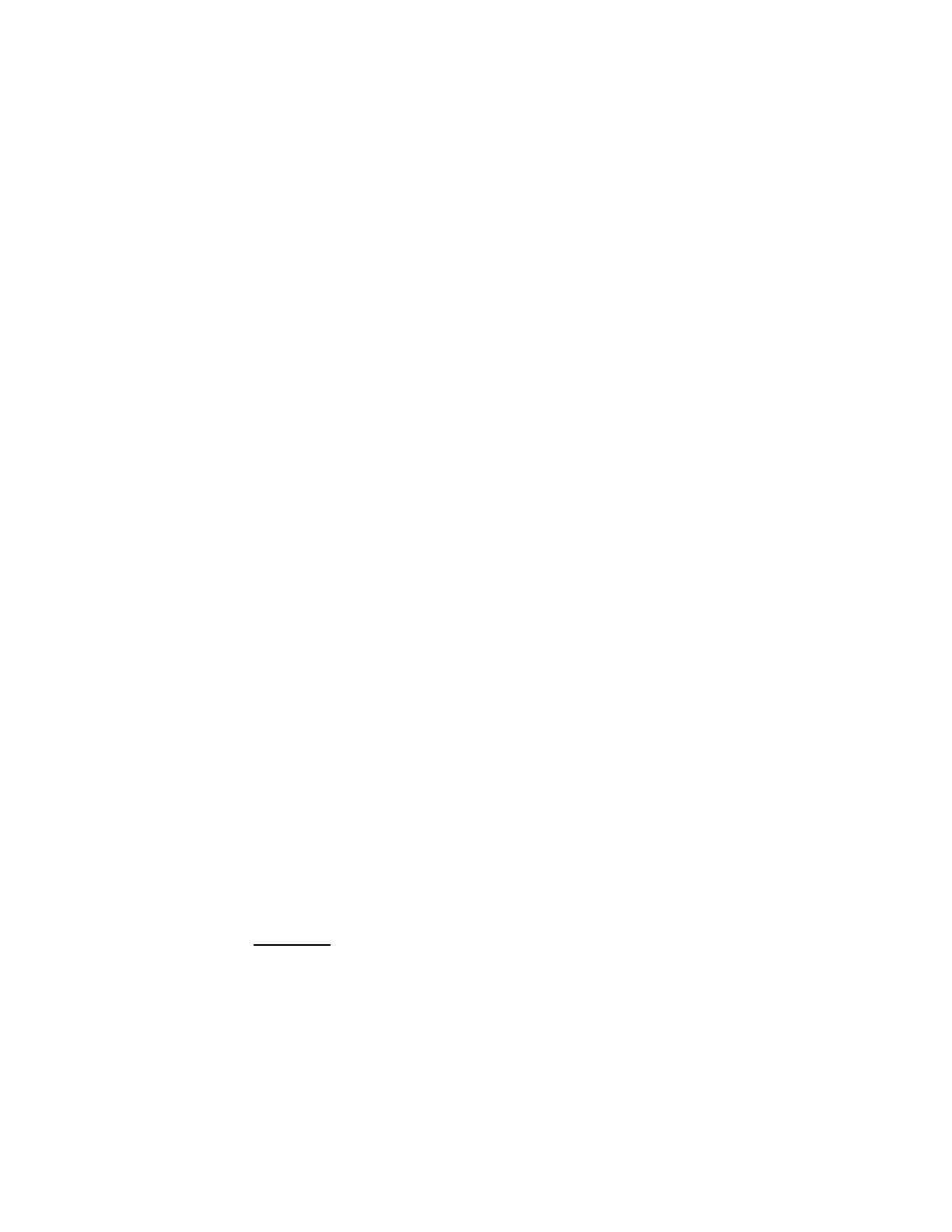
iv
nombre dans le cœur du noyau accumbens des animaux 6-OHDA lésés. Ces
observations, suggérant une régression du double phénotype TH/VGLUT2 avec l'âge,
sont venues renforcer l'hypothèse d'un rôle particulier d'une co-libération de
glutamate par les neurones mésencéphaliques DA au cours du développement.
Dans ces conditions, il est apparu des plus intéressants d'examiner
l'innervation DA méso-striatale chez deux lignées de souris dont le gène Vglut2 avait
été sélectivement invalidé dans les neurones DA du cerveau, ainsi que leurs témoins
et des souris sauvages. D'autant que malgré l'utilisation croissante de la souris en
neurobiologie, cette innervation DA n'avait jamais fait l'objet d’une caractérisation
systématique en microscopie électronique. En raison de possibles différences entre le
cœur et la coque du noyau accumbens, l'étude a donc porté sur les deux parties de ce
noyau ainsi que le néostriatum et des souris jeunes (P15) et adultes (P70-90) de
chaque lignée, préparées pour l'immunocytochimie de la TH, mais aussi pour le
double marquage TH et VGLUT2, selon le protocole précédemment utilisé chez le
rat.
Les résultats ont surpris. Aux deux âges et quel que soit le génotype, les
terminaisons axonales TH immunoréactives des trois régions sont apparues
comparables quant à leur taille, leur contenu vésiculaire, le pourcentage contenant
une mitochondrie et une très faible incidence synaptique (5% des varicosités, en
moyenne). Ainsi, chez la souris, la régression du double phénotype pourrait être
encore plus précoce que chez le rat, à moins que les deux protéines ne soient très tôt
ségréguées dans des varicosités axonales distinctes des mêmes neurones DA. Ces
données renforcent aussi l’hypothèse d’une transmission diffuse (volumique) et d’un
niveau ambiant de DA comme élément déterminant du fonctionnement du système
mésostriatal DA chez la souris comme chez le rat.
Mots clés : dopamine, glutamate, transporteur vésiculaire du glutamate de
type 2, noyau accumbens, néostriatum, substance noire, terminaisons (varicosités)
axonales, co-localisation, développement, transmission diffuse, immunocytochimie,
double marquage.