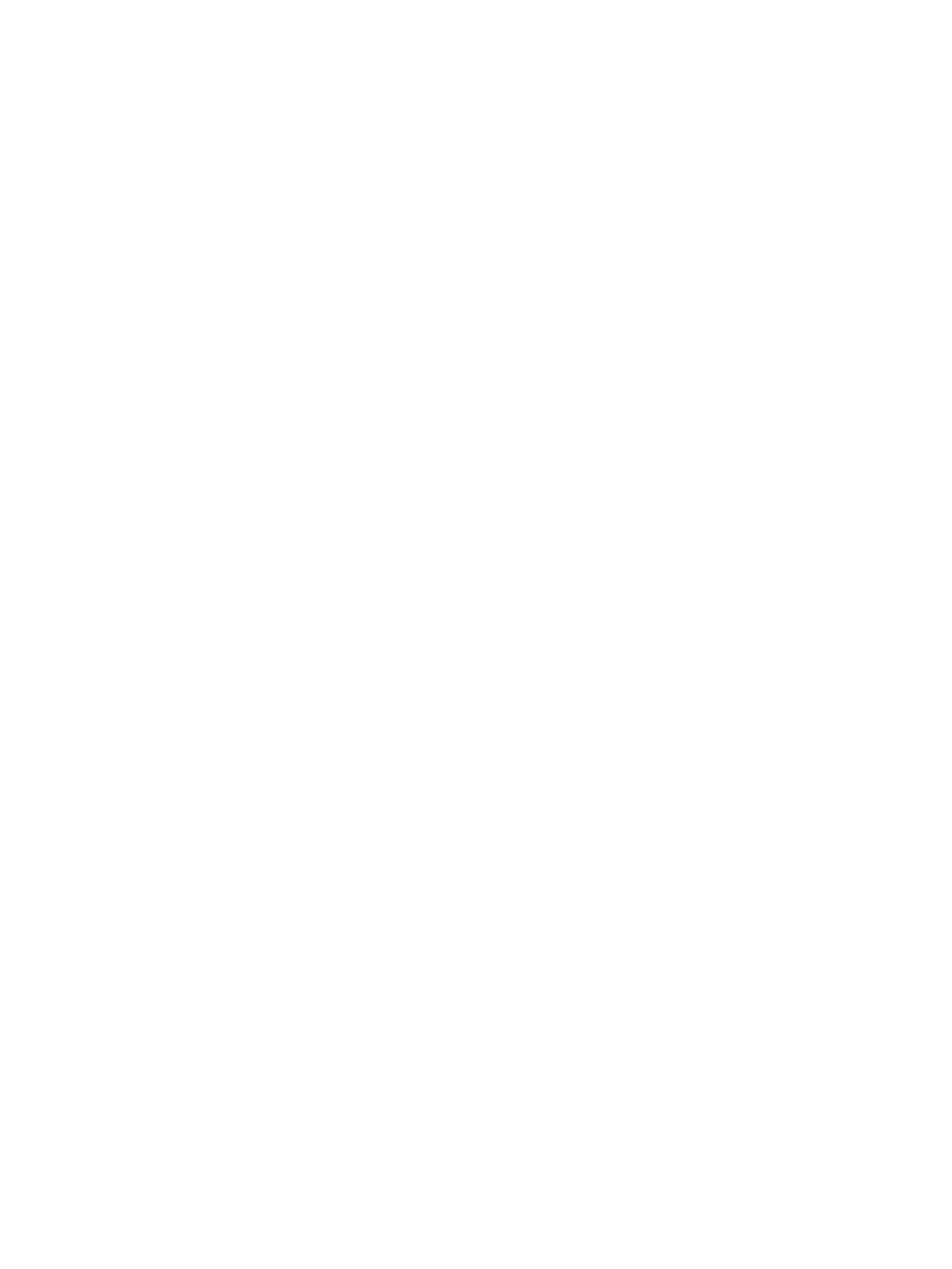
Applied Surface Science 562 (2021) 150161
2
electrocatalyst by polarizing the C atoms given its better electronegative
value than carbon and its provision of more active sites [28], in addition
to the easy formation of the C
–
N bond by reason of the close proximity
of the N radius to that of C [29]. Previous reports has conrmed that
nitrogen doping on carbon nanostructures lowers the anti-bonding
density of states (DOS) of the adsorbed hydrogen, this in turn, reduces
the HER activation energy [30]. Thus metal nanoparticles or their alloys
encapsulated in nitrogen-doped carbon nanotubes exhibits an overall
improvement in their electrocatalytic activity [31,32]. Owning to the
advantages of N-doping on nickel encapsulated carbon nanotubes for
HER, researchers such as Oluigbo et al., fabricated NiNCNT with an
overpotential of 244 mV and Tafel slope of 93.3 mV dec
-1
at 10 mA cm
−2
in 1 M KOH solution [11]. Wang et al., had synthesized Ni@NCNT for
HER that afforded an overpotential of 256 mV and Tafel slope of 131.8
mV dec
-1
at 10 mA cm
−2
in 1 M KOH solution [33]. In addition, Ni/N-
CNT HER electrocatalyst prepared by Jia et al., in 1 M alkaline solu-
tion obtained
η
10
greater than 200 mV and a Tafel slope of 141 mV dec
-1
[34]. While the N-CNT/Ni HER electrocatalyst fabricated by Wang et al.,
in 1 M KOH electrolyte at 10 mA cm
−2
, afforded a value of 308 mV as
overpotential and a Tafel slope of 140.2 mV dec
-1
[35]. However,
preparation of the CNT requires CVD method which is expensive with
complex design of reactors. Therefore, in-situ fabrication of N-doped
NiCNT provides the advantages of a facile, eco-friendly, cheap and less
time-consuming synthetic pathway for efcient, highly conducting and
stable HER electrocatalysts.
In this work, a simplistic and in-situ route is employed in the fabri-
cation of nitrogen and nickel carbon nanotubes. In addition, the cata-
lysts are realized in a small time of 2 h and requires no rigorous post
treatments. The synthesis also involves melamine as a nitrogen and
reducing agent, nickel acetate and glucose as nickel and carbon sources
respectively. As the annealing process progressed, nickel ions (Ni
2+
)
were transmuted to metallic nickel by the reducing species from mel-
amine which then catalysed the formation of bamboo-like structured
CNT. The fabricated electrocatalyst portrayed outstanding performance
towards HER. Furthermore, the DFT calculations of the HOMO-LUMO
energy gap, ionization potential and adsorption energies furnished
more insight on the electrochemical performance of the electrocatalysts
towards HER.
2. Experimental section
2.1. Chemicals
Potassium hydroxide (KOH) (≥85.0%), glucose D (C
6
H
12
O
6
⋅H
2
O)
(≥98.0%), nickel (II) acetate tetrahydrate (NiC
4
H
6
O
4
⋅4H
2
O) (≥98.0%),
absolute ethanol (C
2
H
5
OH) (≥99.5%) and melamine (C
3
H
6
N
6
)
(≥99.0%) obtained from Sinopharm chemical reagent Co., Ltd. The
continuous carbon/formvar support lm was purchased from Zhong-
jingkeyi (Beijing) Film Technology Co., Ltd.
2.2. Fabrication of nickel-nitrogen doped CNT
In a conventional procedure, Nickel (II) acetate tetrahydrate (2
mmol) and Glucose (2 mmol) were added to 20 mL aqueous solution of
melamine (40 mmol) which was kept stirring for 2 h at 80 ◦C. The
mixture, dried for 48 h at 80 ◦C was comminuted with an agate mortar
and pyrolyzed for 2 h at temperatures of 750, 800 and 850 ◦C and ramp
rate of 2◦min
−1
under a stream of N
2
. The samples obtained after being
thoroughly rinsed with water and absolute ethanol were placed in an
electric oven at 70 ◦C to dry. The samples were then labelled as Ni-
NCNT
7.5
, Ni-NCNT
8.0
and Ni-NCNT
8.5
in correspondence with the py-
rolysis temperatures.
2.3. Physical characterization
To investigate the crystal structure and phase purity of the samples
by X-ray diffraction (XRD), a D8 Advance X-ray diffractometer [Bruker
AXS Company, Germany] was employed. A DXR laser Raman spec-
trometer [Thermo Fisher Corporation, USA] using a 532 nm laser source
was employed in obtaining the Raman spectra at room temperature. X-
ray photoelectron spectrometer (PHI-500 Al K
α
radiation) was
employed to record the chemical states of the elements present. For the
purpose of probing the morphology, composition and particle size of the
samples, scanning electron images (SEM), energy dispersive X-ray
spectroscopy (EDX) and elemental mapping images were captured with
a [Hitachi S-4800 II, Japan] microscope operating at 15.0 kV. Trans-
mission electron (TEM) and high-resolution transmission electron (HR-
TEM) images were measured on a [JEOL-JEM-2010 JEOL, Japan]. The
pore structure was probed with a surface analyser (Micrometrics, ASAP
2020 HD88) by determining the Brunauer-Emette-Teller (BET) specic
surface area and pore size distribution from the BET and Barrett-Joyner-
Halenda (BJH) models. The nickel content was determined by induc-
tively coupled plasma-optical emission spectroscopy (ICP-OES) with a
Thermo Scientic iCAP 7000.
2.4. Electrochemical characterization
All the electrochemical measurement was investigated on a 660D
electrochemical analyser with the traditional three-electrode arrange-
ment at 25 ◦C in a 1 M potassium hydroxide N
2
-saturated solution.
Carbon rod, Hg/HgO and modied glassy carbon electrode (GCE 3 mm
diameter) were used as the counter, reference and working electrodes,
respectively. The GCE was modied by dropping 5 µL of a homogenous
catalyst ink on its polished surface and left to dry at an ambient atmo-
sphere. The catalyst ink was readied by a 40 min ultrasonication of the
catalyst (5 mg), naon (30 µL) (5 wt%), absolute ethanol (250 µL) and
deionised water (720 µL) mixture. Consequently, the loaded mass of the
catalyst on the GCE was 0.357 mg cm
−2
and was activated by per-
forming 50 CV cycles. A 5 mV s
−1
scan rate and a potential limit of 0.124
to −0.476 V (vs RHE) were employed to record the polarization (LSV)
curves. With similar conditions, commercial Pt/C (10 wt%) was applied
for comparison. All the potentials were IR-corrected and translated to
the reversible hydrogen electrode (RHE) by the formula: E
RHE
=E
vs Hg/
HgO
+E
ϴHg/HgO
+0.059 pH. The electrochemical impedance spectros-
copy (EIS) was recorded by open circuit potentials with an amplitude of
5 mV in the frequency limit of 1.0 to 10
5
Hz. A potential value of −0.376
V vs RHE was applied in the chronoamperometry (i.t) experiment for a
20 h stability. For the electrochemically active surface area (ECSA),
double layer capacitances (C
dl
) were recorded from CV scans using scan
rates from 40 to 160 mV s
−1
. The C
dl
values were obtained from the
slopes of the graph of Δj at 0.1 V vs RHE against the scan rates.
2.5. Computational method
The density functional theory (DFT) calculations was carried out to
optimize the geometry of carbon nano-tube (CNT) using DMol3 code in
the Material Studio Software [36]. The CNT was imported from material
studio structural data collection and was modelled using the build
nanotube interface. The electronic structures of CNT were studied with
generalized gradient approximation (GGA) and Perdew-Burke-
Ernzerhof (PBE) exchange–correlation function including dispersion
corrections (DFT-D) [36,37]. The calculations were spin restricted with
displacement and convergence maximum force at 0.005 Å and 0.004
Ha/ Å respectively. The armchair CNT was only considered because it
has enhanced charge transport features which corresponds with the
experimental ndings of our studies and has been widely used to
simulate CNT [38,39]. A total of 8 congurations of the arm-chair car-
bon nanotubes were optimized. The optimized armchair nanotube was
further doped with nitrogen and nickel atoms by replacing a carbon
atom with a nitrogen or nickel atom in the middle side wall of the carbon
nanotubes. The bond lengths, bond angles, and the HOMO-LUMO en-
ergy gap were calculated. The adsorption of water molecule on the
C.J. Oluigbo et al.