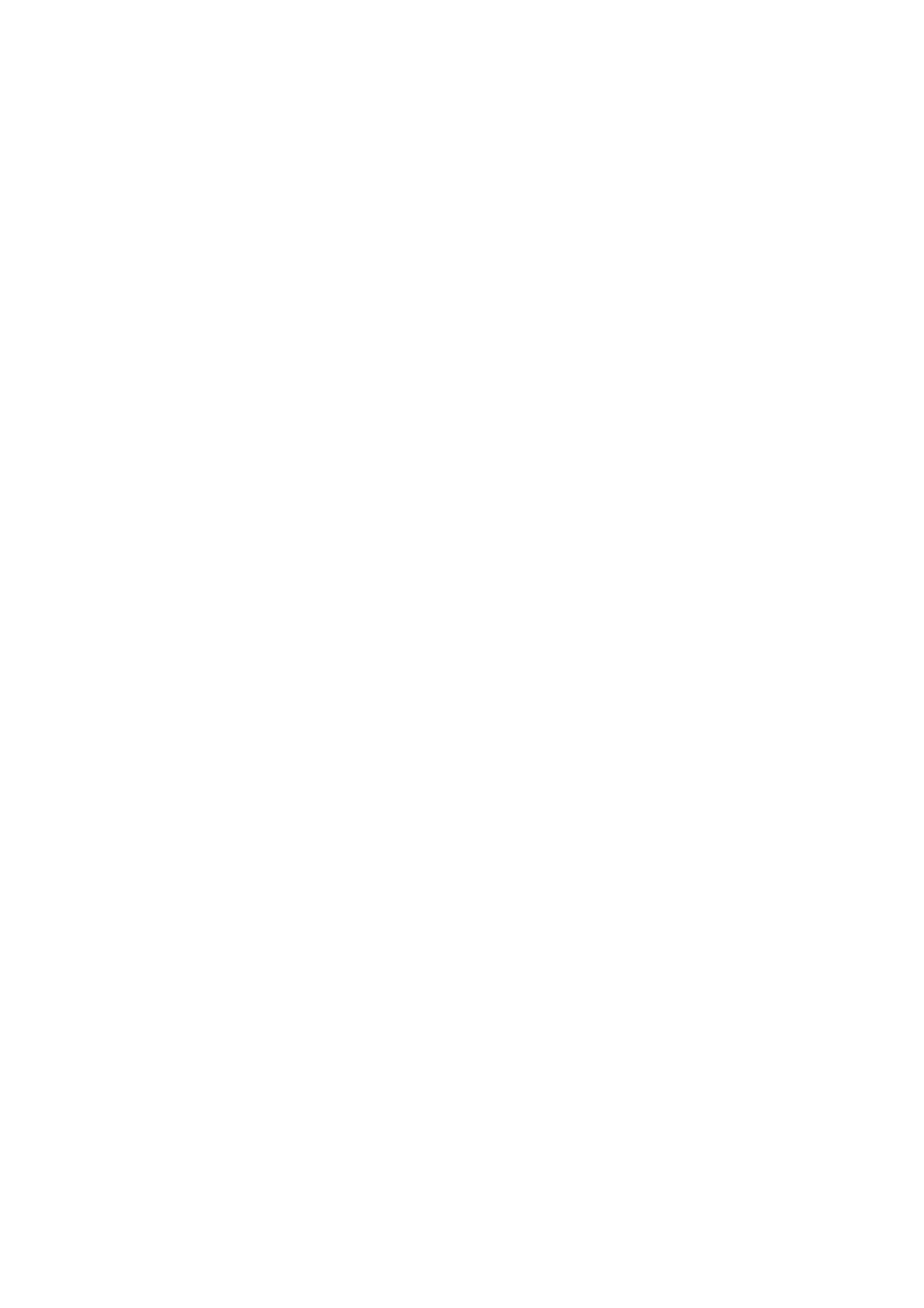
247
switched two-inductor boost converter or by varying the switching frequency in the
soft-switched two-inductor boost converter. However, the two-inductor boost
converter is a boost derived converter and zero output voltage cannot be reached in
either the hard-switched or the soft-switched forms. In order to generate the
rectified sinusoidal waveforms at the output of the two-inductor boost converter, a
buck conversion stage must be added. Therefore the converters in Figures 3.10 and
3.11 can be developed.
Recently, multi-phase converter arrangements have been widely adopted as an
efficient approach to parallel multiple converters to provide high current output
[168]. Under multi-phase operation, the individual converter input and output
currents with an equal phase shift, which is the quotient of 360º divided by the
number of phases, are added together and the equivalent input and output current
ripple frequencies will be multiplied by the number of the phases. The converter
also has a smaller input or output current ripple magnitude as the current ripples in
the individual phases cancel [169]. This eases the requirement on bulky input and
output filter components such as inductors and capacitors. A two-phase buck
converter will be employed as the current source for the two-inductor boost
converter.
In order to feed the output from the two-phase buck converter to the input of the
two-inductor boost converter and make use of the two existing inductors in the boost
converter, an interphase transformer (IPT) is utilised. The IPT is a tapped inductor,
which has 1:1 turns ratio. The IPT has been previously used in the dc-dc converter