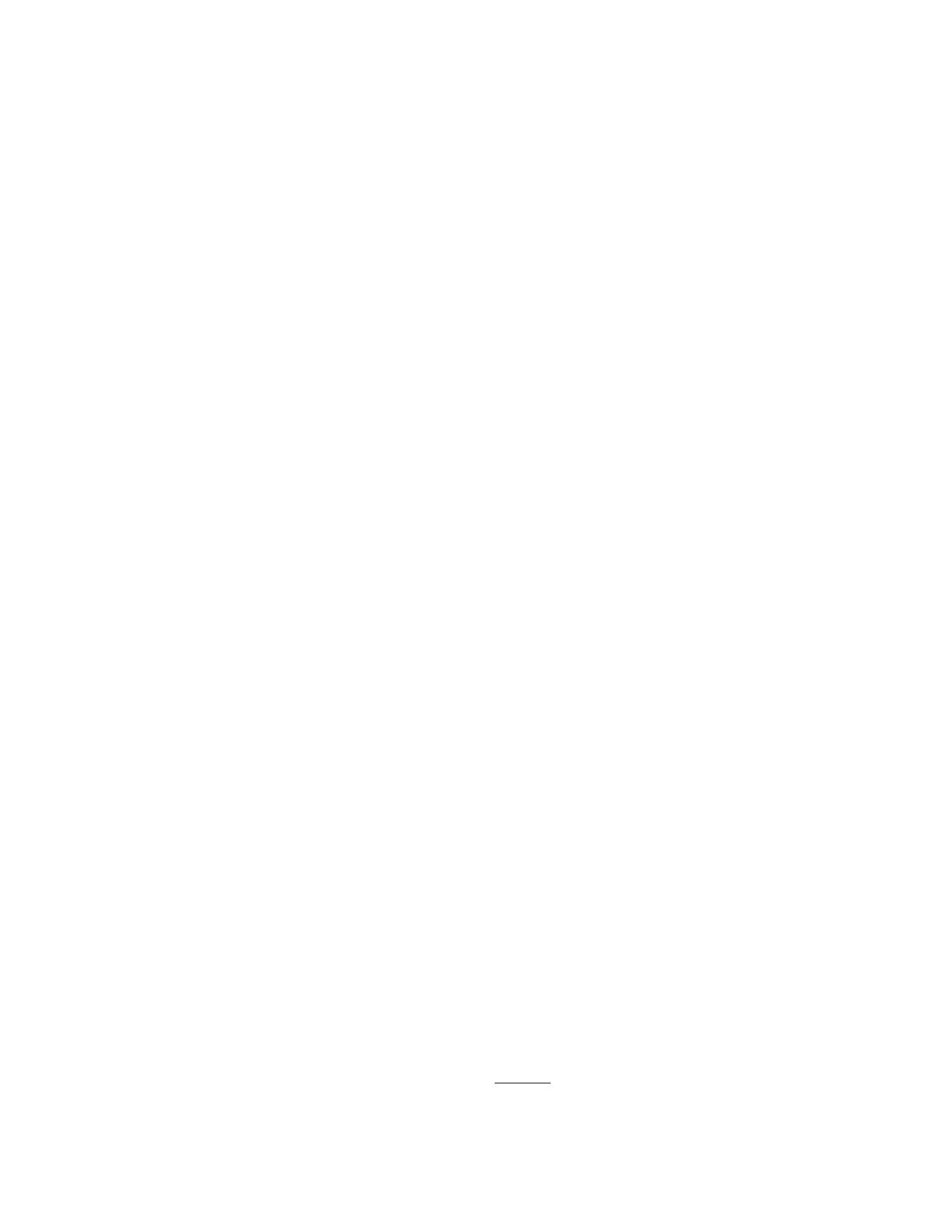
between the stadia rod and the alidade. Chaining pins are not
required when this method is used. In practice, more readings
closely spaced along the vegetational outline usually are taken
using the basepoint-stadia rod-alidade method.
It is not necessary to measure all distances and angles; some
can be determined trigonometrically. After all angles and dis-
tances are calculated, fill in irregularities via inspection and use
of other maps and photographs.
The technology for using global positioning systems (GPS),
especially when linked to geographical information systems
(GIS) and remote sensing, is evolving rapidly.
2
These systems
have wide applicability to mapping aquatic vegetation, thereby
rendering older surveying techniques obsolete or at least time-
consuming and tedious.
2.
Line Intercept Method
The line intercept method is preferable for mapping mixed
stands and/or large areas. Select sampling points at equal inter-
vals along a baseline. Choose interval length by the degree of
accuracy desired: the closer the sampling points, the more accu-
rate the map. Run transects perpendicularly from the baseline to
the boundary of the plant stand. Use an intercept line (transect
line) of plastic-coated wire rope to prevent stretching. If line
flotation is a problem, use lead weights applied at regular inter-
vals to sink the line and act as interval markers on the rope to
designate sampling units. Use 0.5-m (in dense vegetation) to 5-m
sampling units (in sparse vegetation) for determining plant spe-
cies that vertically intercept the line at each segment. During
underwater surveys, record data with a wax or soft lead pencil on
a writing board constructed of plastic overlays. Construct the
vegetation map by placing points where plant species are found
within an outline map (or aerial photograph) of the sample area.
Determine the area that a single species or total vegetation
occupies by planimetry or digitization and computer calculation.
Determine frequency from line intercept or quadrat data, or with
a set sampler consisting of a 2-cm steel tube, 2 m long, to which five
0.75-cm by 25-cm steel rods are attached on 40-cm centers. Record
vegetation touching each of the five points within 2.5 cm of the
distal tip. If more than one plant species is touching, record the plant
nearest the tip. If no plant is touched, record bare ground.
3.
Remote Sensing
a. General considerations: Remote sensing techniques are
used to detect, assess, and monitor aquatic macrophytes. These
techniques include analog aircraft and satellite serial photogra-
phy, digital aircraft and satellite multispectral scanning in the
visible, infrared, and thermal bands; microwave techniques, pri-
marily side-looking airborne radar (SLAR); and shuttle imaging
radar (SIR). They provide a synoptic view of large areas, and
allow quick surveys to further delineate areas of interest and
repeated viewing at relatively low cost.
In selecting remote sensing system(s), consider expected re-
sults, time for project completion, and available resources. The
larger the area, the greater the advantage of remote sensing.
Remote sensing also lends itself to studies over time, because
each image is a historical record.
For determining general associations of a widespread macro-
phytic growth, ground resolutions of between 30 and 80 m are
recommended. Widespread multitemporal coverage is available
at scales ranging from 1:100 000 to 1:1 000 000 at a reasonable
cost in the form of paper prints, transparencies, and digital
formats. Landsat Multispectral Scanner (MSS) and Thematic
Mapper (TM) have been used to identify areas with emergent
vegetation or topped-out submergent vegetation. Surface rough-
ness is a requisite for an imaging return with SLAR and SIR.
Landsat provides limited capability for species discrimina-
tion, but availability, cost, and repeat cycles make it useful in
determining the presence of large populations over time. For
a detailed vegetation survey, including discrete species iden-
tification, use much larger-scale imagery, (1:10 000 or greater).
High-altitude aerial coverage available through the National
High Altitude Mapping Program (NHAP) and other sources at
original scales of approximately 1:60 000 to 1:120 000 provides
both good initial areal coverage and the capacity for enlargement up
to five times.
3
After determining scale, select film/filter or sensor combinations.
These include black and white imagery, color infrared, and black
and white-infrared imagery; color infrared film used with a yellow
filter is widely applicable, but other combinations also are useful.
4–7
For detailed flight planning, consider growth stage of plants, water
depth and clarity, tidal conditions, cloud cover, and sun angle.
3,8,9
Available resources ultimately determine remote sensing activities.
b. Aerial photography:
1) Equipment—For all-format aerial photography, use a
good-quality 35-mm single-lens reflex (SLR) camera with man-
ual or through-the-lens metering or any good 70-mm camera
system, preferably with a motor drive. Intervalometers providing
for exact exposure intervals for stereo photography are available
for both systems. A 28-mm-focal-length lens with a 35-mm SLR
camera gives wide coverage at low altitudes; 40-mm and 80-mm
lenses are successful with 70-mm camera systems. When pho-
tographing with black-and-white or color film, use a skylight or
haze filter. When photographing with color infrared film below
1700 m, use a Wratten 12 filter; at altitudes above 1700 m use a
Wratten 15 filter. Time of year and condition of vegetation also
may influence the choice of yellow/orange filter. In turbid wa-
ters, color film* provides better water penetration and is more
useful in mapping submersed vegetation than is color infrared
film.
4
Color infrared† yields more detail, is more useful in
mapping emergent vegetation or wetlands, and may provide
more detail in clear, nonturbid waters. Video cameras (camcord-
ers) and digital cameras also are being used to obtain aerial
photos of vegetation.
Preferably mount camera in the belly of a high-wing, single-
engine aircraft for low-altitude, small-format photography. Belly
mounts require special aircraft modification, but provide a stable
platform, camera protection, and good access. Alternatively,
camera may be mounted on the aircraft door.
2) Procedures—Because sun angle is critical in obtaining
high-quality aerial photography, schedule flight for a time when
solar altitude is between 40 and 68 degrees.
7
Set camera at designated ISO reading for the film (assume 100 to
125 for color infrared film) and shoot in the automatic exposure
mode. At a typical airspeed of about 190 km/h (approximately
* Kodak Ektachrome Professional film type 5036, or equivalent.
† Kodak Aerochrome Infrared film type 2443, or equivalent.
MACROPHYTES (10400)/Vegetation Mapping Methods
3
MACROPHYTES (10400)/Vegetation Mapping Methods