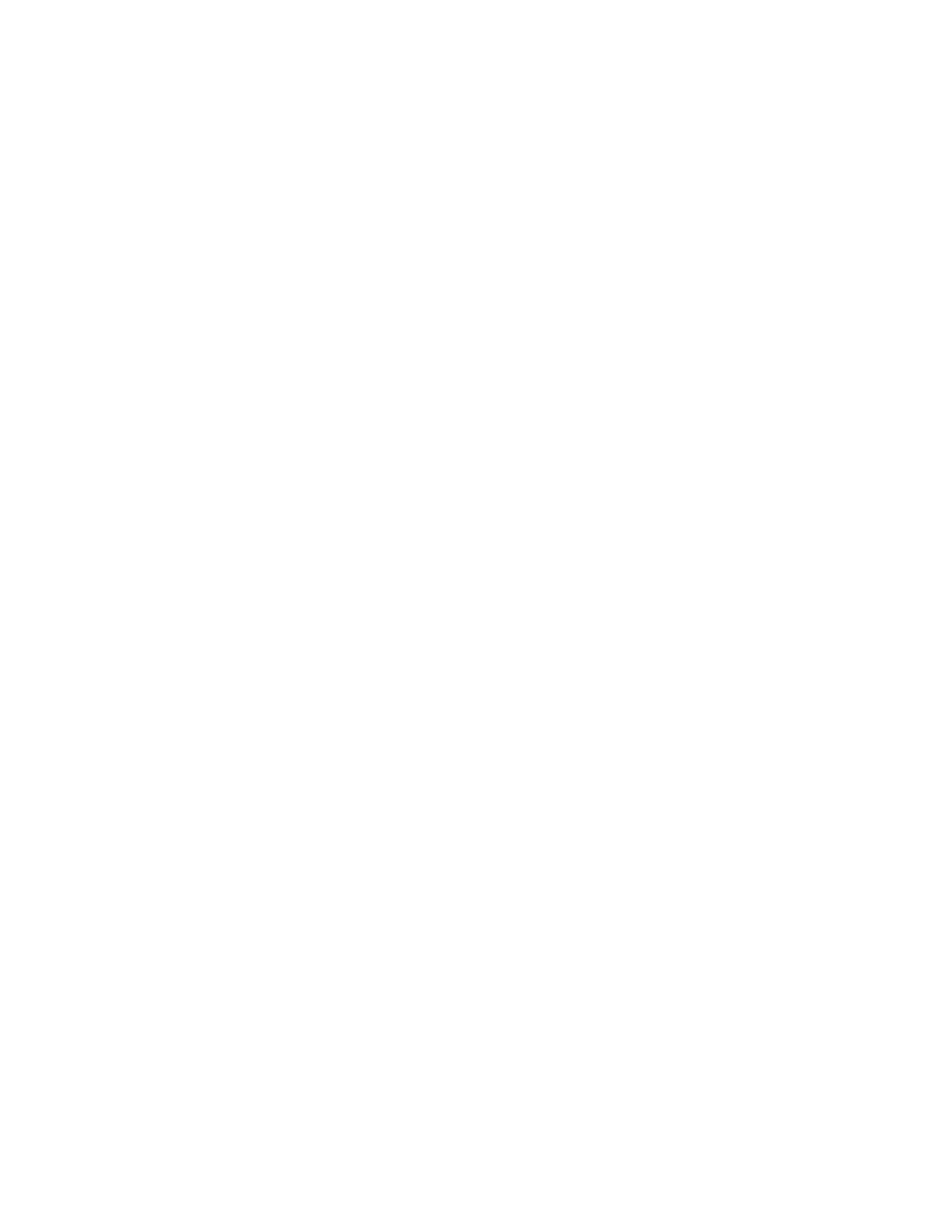
(e.g., to ⬃0.1%) will decrease the magnitude of crosstalk cor-
rections and improve the quality of simultaneous alpha and beta
measurements.
Once the operating voltage and discriminator settings have
been established, they must be documented and locked down.
Changing either parameter invalidates all previously established
performance check data and instrument calibrations, and the
instrument will have to be recalibrated.
4) Background (alpha or beta)—Most detectors have a back-
ground count rate that is due to several factors: cosmic radiation;
radionuclides contained in instrument materials; the counting
room’s construction material; electrical/electronic noise; and/or
the proximity of radioactive sources. The background is roughly
proportional to the size or mass of the counting chamber or
detector but can be reduced by shielding or anti-coincidence
guard circuitry (see 7030B.1d).
a) Determination of background count rate—The background
count rate is subtracted from each measurement to determine the
“net” activity of the sample test source. Determine the back-
ground count rate by counting an empty planchet in the counting
chamber in the same configuration as the sample test source. The
background count duration should be as long as or longer than
the counts from which it will be subtracted.
b) Control of backgrounds—Verify the continuing stability of
the background count rate during the count by tracking back-
ground performance via control charts. Also, evaluate method
blank control charts for persistent high or low bias in results (i.e.,
absolute bias), then determine the cause and correct it. If the
cause is intrinsic to the method, a correction factor (independent
of QC samples) may be developed and applied to eliminate
absolute bias as long as the additional correction’s uncertainty is
reflected in the combined standard uncertainty reported for the
sample result.
5) Initial calibration (alpha, beta, or radionuclide specific)—
The purpose of initial calibration is to empirically derive count-
ing efficiency factors for a geometry that matches those of the
test samples, thus ensuring that the correction for efficiency is
accurate. Parameters that must match include radionuclide, ma-
trix composition, and density/thickness (which causes self-ab-
sorption of alpha or beta particles in the sample matrix). Each
detector’s detection efficiency must be determined separately.
Initial calibration is procedure-dependent; see individual proce-
dures for specific instructions. Absorption curve values do not
need to be re-established after initial calibration as long as
continuing calibration (source check) response is monitored reg-
ularly and indicates that the instrument has remained stable
between calibration and sample measurements.
6) Continuing calibration (source check)—Verify instrument
stability at the operating voltage by counting separate check
sources for alpha and beta (where applicable) on each detector.
Monitor the major and minor channel count rates (i.e., for an
alpha source, alpha is the major channel and beta is the minor
channel) for each source counted and plot the results on a
control/tolerance chart to demonstrate the continuing stability of
the detector efficiency and crosstalk (see Section 7020A). The
tests and acceptance criteria used should be addressed in the
laboratory quality manual. If the checks do not provide evidence
of continuing instrument stability, the detector should be taken
offline until the problem is fixed and the instrument is recali-
brated, or until continuing checks demonstrate that maintenance
has not changed instrument response.
7) Sample counting—Place the prepared sample test source in
the detector in a geometry consistent with initial calibration. For
internal GPCs, ensure that there is electrical contact between
planchet and chamber, and flush the chamber with counting gas.
Count for a preset duration or a preset count to give the desired
counting uncertainty (see Section 7020D).
2.
Alpha Scintillation Counter
Alpha scintillation counters detect and quantify alpha-emitting
radionuclides. If the sample contains multiple radionuclides, the
instrument will detect and count all alpha emissions regardless of
their radionuclide source or energy. The analysis may be made
more radionuclide-specific by using a radiochemical separation
to isolate the desired radionuclide. This radionuclide (
226
Ra, Th,
U, etc.) is usually precipitated and mounted as a thin layer
(ⱕ5 mg/cm
2
) on planchets. Radon-222 (radium-226 by radon
emanation) also can be counted in alpha scintillation cells in a
modified sample chamber (see Section 7500-Ra.C).
a. Principle and uses: An alpha particle interacts with zinc
sulfide phosphor (which contains a silver activator), exciting the
scintillator’s atoms. When the atoms return to the ground state,
they emit the energy as visible light. This process is called
scintillation. The light is further transformed into an electrical
current via an attached PMT, which amplifies the electrical
current into a measurable pulse. The pulses trigger a scaler,
which registers each pulse as a “count.” Depending on the
amount of radionuclide present and statistics required, count a
sample long enough to obtain required sensitivity. The counter is
calibrated with a thin-layered precipitate of a radionuclide or an
electrodeposited radionuclide.
b. Components: The alpha scintillation counter consists of a
light-tight sample chamber with a phosphor detector coupled to
a photomultiplier tube (PMT) and sample holder, a high-voltage
supply, an amplifier-discriminator, a scaler, and readout capabil-
ity. Generally, the PMT’s window has a larger diameter than that
of the samples. Put the phosphor between the sample and the
PMT. The distance between the sample surface and PMT face is
usually about 3 to 5 mm. Arrange the phosphor so the PMT
window is in optical contact with the scintillator. Under these
conditions, the counting efficiency can be 35 to 40%. For details
on operating and calibrating an alpha scintillation counter, see
manufacturer’s instructions.
c. Performance verification:
1) Radioactive source—See Section 7020A.3h.
2) Plateau—In accordance with manufacturer recommenda-
tions, use a radioactive source to find the operating voltage
where the count rate is consistent over some specified voltage
range.
3) Background—Two backgrounds are evaluated with alpha
scintillation counters: electronic instrument background and
chamber background. The electronic instrument background
characteristically measures the PMT’s electronic noise. It is
determined periodically (e.g., annually) by counting with an
empty chamber and should vary from 0 to 1 cpm.
The chamber background is counted with the zinc sulfide
phosphor or scintillation cell in place. This background is caused
by contaminated instrument parts, counting-room construction
COUNTING INSTRUMENTS (7030)/Description and Operation of Instruments
3
COUNTING INSTRUMENTS (7030)/Description and Operation of Instruments