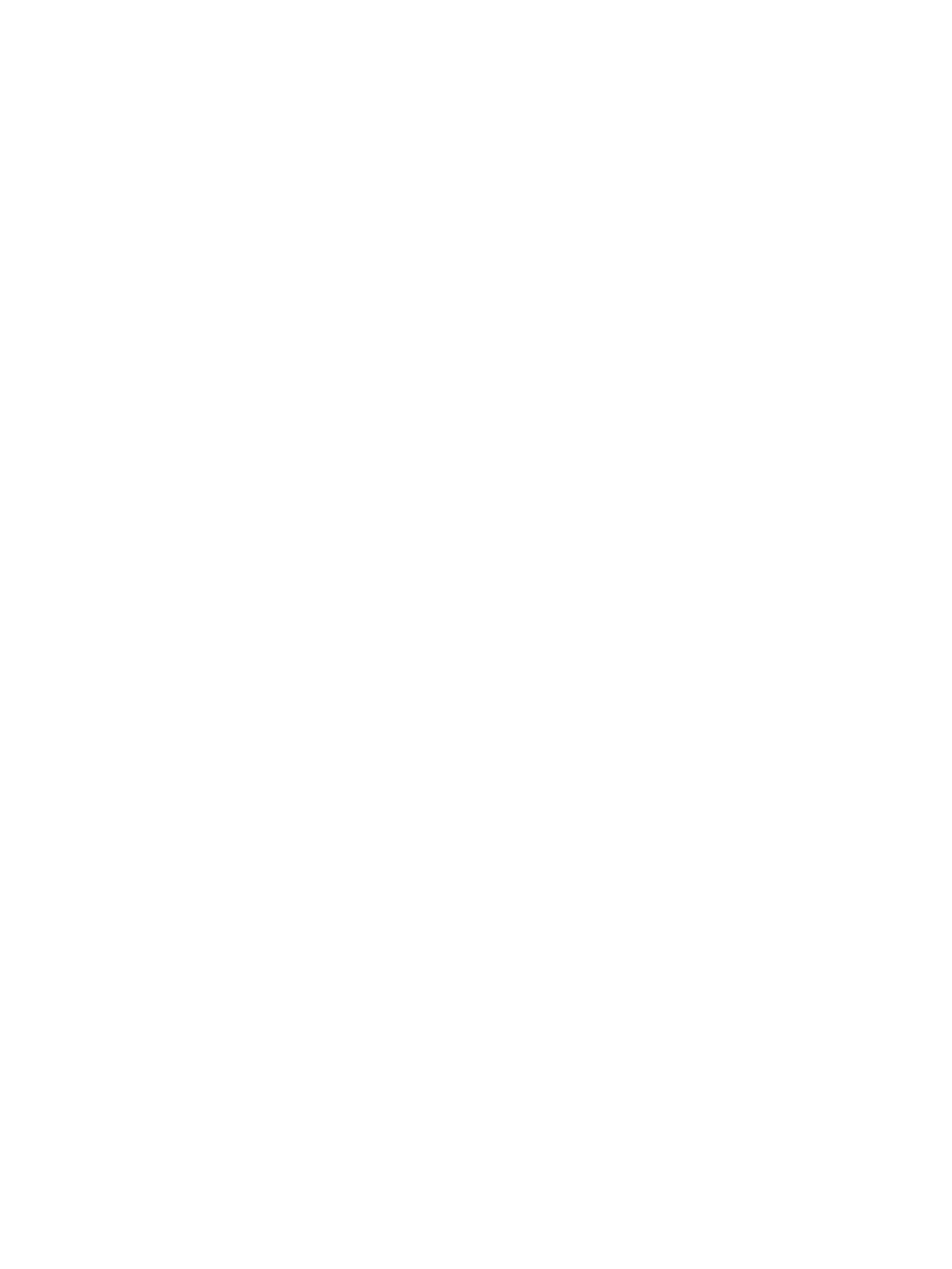
2HO
2
!O
2
+H
2
O
2
ð9Þ
Therefore catalytic ozonation process can be used to enhance
the degradation efficiency of organic pollutants, including homo-
geneous and heterogeneous catalytic ozonation.
In homogeneous catalytic ozonation process, liquid catalysts,
especially transition metal ions are used, such as Fe
2+
,Mn
2+
,Ni
2+
,
Co
2+
,Cd
2+
,Cu
2+
,Ag
+
,Cr
3+
,Zn
2+
in reaction solution. These catalysts
can excite ozone to generate hydroxyl radicals (
OH) and improve
degradation efficiency.
In heterogeneous catalytic ozonation process, solid catalysts
such as metal oxide, activated carbon, porous materials and their
composite materials are added into reaction solution (Kasprzyk-
Hordern et al., 2003).
3.1. Ozone concentration
The ozone concentration has important influence on the degra-
dation of antibiotics. The mass transfer rate and the volumetric
mass transfer coefficient of ozone increases with increase of ozone
concentration. More ozone can be absorbed and react with antibi-
otic molecules, finally improving the decomposition of antibiotics
(Zhao et al., 2006; Kornmuller and Wiesmann, 2003).
Oh et al. (2016) studied the influence of ozone dosage on degra-
dation of antibitics, they found that tetracycline was degraded
more quickly at 7 ppm ozone exposure than at 3 ppm. Iakovides
et al. (2019) found that the elimination of antibiotics increased
when the ozone dosage increased, including ampicillin, azithromy-
cin, clarithromycin, erythromycin, ofloxacin, sulfamethoxazole,
tetracycline, trimethoprim. Paucar et al. (2019) studied the degra-
dation of ciprofloxacin, levofloxacin, clarithromycin and nalidixic
acid, they found that antibiotics degradation enhanced when the
initial ozone concentration increased. Hollender et al. (2009)
explored the effect of ozone dosage on the elimination of various
micro-pollutants. Overall, the removal efficiency of selected
micro-pollutants increased with increase of ozone dosage. De
Witte et al. (2009) investigated the ozonation of ciprofloxacin,
and found that the pseudo first-order constants increased with
the increase of ozone inlet concentration.
3.2. pH value
Generally, ozone can degrade organic pollutants through direct
oxidation by ozone molecule in acidic condition. In alkaline condi-
tion, organic pollutants are oxidized by both ozone molecule and
hydroxyl radicals (
OH) (Ikehata et al., 2006; Yargeau and Leclair,
2008). Thus, the degradation of antibiotics by ozonation depends
on the solution pH values.
Feng et al. (2016a) found that the degradation of flumequine
was faster at higher pH values. The reaction rate constant
increased from 0.3772 min
1
to 2.5219 min
1
when pH values
increased from 3.0 to 11.0. In alkaline condition, more O
3
was
transformed to
OH, and indirect oxidation of
OH could be more
beneficial in decomposition of flumequine than direct oxidation
of O
3
. Moreover, the species of flumequine under different pH also
influenced the result.
Wang et al. (2012) explored the chloramphenicol (CAP) degra-
dation by ozone in aqueous solution at various initial pH values.
The removal efficiency of chloramphenicol (CAP) was 41.4 ± 1.0%
and 65.3 ± 3.0%, respectively at initial pH of 2.0 and 8.0. This result
may be attributed to more free radicals generated in alkaline con-
ditions. However, the removal rate decreased at initial pH of 10.0.
Oncu and Balcioglu (2013) investigated the influence of pH on
the ozonation of ciprofloxacin (CIP) and oxytetracycline (OTC).
Higher degradation of ciprofloxacin (CIP) and oxytetracycline
(OTC) was achieved at higher pH.
Jung et al. (2012) examined the effect of pH on the degradation
efficiency of ampicillin, the biodegradability and toxicity after
ozonation. The second-order rate constant and COD removal rate
increased with increase of pH when pH was in the range of 5–9.
A higher biodegradability and acute toxicity was observed at the
highest pH (pH 9).
On the one hand, an increase of ozone decomposition to gener-
ate
OH occurred in alkaline condition. On the other hand, non-
protonated organic amine species (–NH
2
) was more reactive
toward ozone molecules than the mono-protonated form (–NH
3
)
(Hoigne & Bader, 1983). At pH 9, non-protonated amine (–NH
2
)
was the dominant group of ampicillin, which could be attacked
by ozone more easily.
3.3. Mineralization of pollutants
Usually, the ozonation process could not totally mineralize the
antibiotics. On the one hand, carbonate (CO
3
2–
) and bicarbonate
(HCO
3
–
) formed during antibiotic decomposition process are hydro-
xyl radical scavengers, which can inhibit the removal of antibiotics.
On the other hand, solution pH decreased with the ozonation reac-
tion proceeding, which is adverse for the generation of hydroxyl
radicals (
OH). Uslu and Balcioglu (2008) observed that mineraliza-
tion rate of oxytetracycline reached 20% after 30 min ozonation at
pH 8.5. Feng et al. (2016a) found that 39.45% of TOC was removed
after the ozonation of flumequine aqueous solution. Kuang et al.
(2013) observed complete trimethoprim degradation after ozona-
tion, while no mineralization was determined. Goncalves et al.
(2012) found that TOC removal efficiency was 33.5% after
180 min ozonation of sulfamethoxazole solution.
3.4. Biodegradability improvement of pollutants
The BOD
5
/COD ratio is usually used for characterizing the
biodegradability of a pollutant or wastewater. The biodegradability
of antibiotics wastewater can be improved by ozonation due to the
generation of low molecule weight and biodegradable intermedi-
ate products.
Balcioglu and Otker (2003) observed that biodegradability of
wastewater after ozonation increased. More low-molecular weight
intermediate products that are more amenable to biodegradation
generated after ozonation (Stockinger et al., 1995).
Jung et al. (2012) found that the BOD
5
/COD ratio at 9 increased
constantly from 0 to 0.41 after 120 min of ozonation, enhancing
the biodegradability and biological treatability of ampicillin-
containing wastewater.
Dantas et al. (2008) reported that the biodegradability
increased from 0 to 0.3 during sulfamethoxazole ozonation, indi-
cating that the antibiotic was conversed to biodegradable interme-
diate product.
Uslu and Balcioglu (2008) observed that the BOD
5
/COD ratio of
synthetic oxytetracycline wastewater increased from 0.05 to 0.3
due to the formation of biodegradable intermediate products dur-
ing ozonation process.
3.5. Antibiotics removal by ozone oxidation
The degradation of various antibiotics by ozonation was sum-
marized in Table 2.
4. Photocatalytic oxidation
Photocatalytic oxidation has been extensively studied for the
degradation of organic pollutants. Semi-conductor materials, such
as TiO
2
, ZnS, WO
3
and SnO
2
are used as photo-catalyst. When
J. Wang, R. Zhuan / Science of the Total Environment 701 (2020) 135023 5