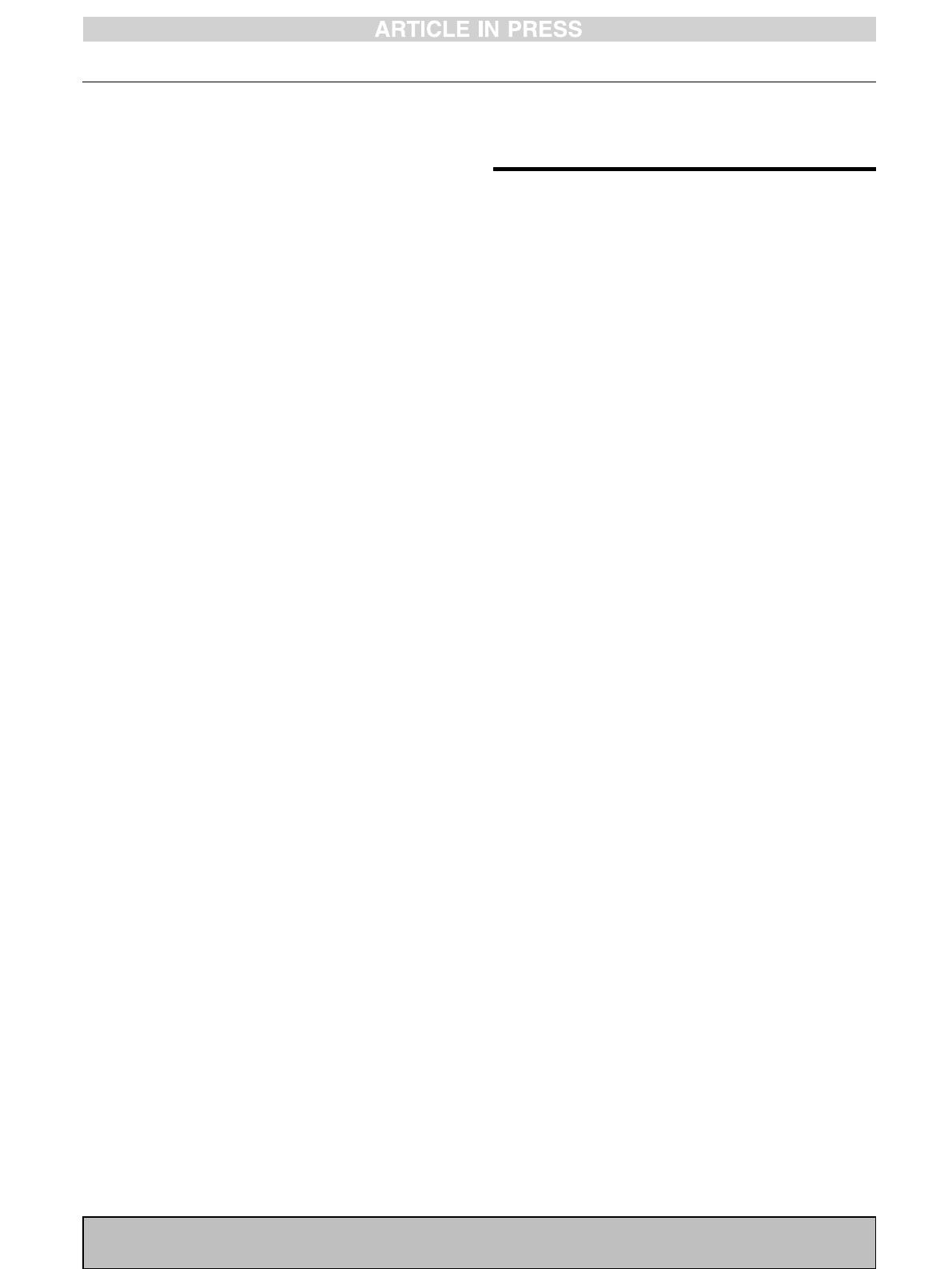
workers [18] thought that the high electrochemical activity for
the hydrogen evolution of the NieMo coating could be attrib-
uted to both exchange current density and larger real elec-
trode area. Navarro-Flores et al. [19] studied the influence of
alloying nickel by Fe, Mo and Won the electrocatalytic activity
towards HER in an acidic environment. They claimed that
NiW, NiMo and NiFe electrodes were more efficient for
hydrogen generation that pure nickel. NieMo was found to
yield the highest overall electrocatalytic activity, mainly due
the highest surface roughness, while NieW yielded the high-
est intrinsic activity as a result of the modification of electron
density in d-orbitals upon alloying nickel with tungsten.
Conway and co-workers [20] investigated the catalytic activity
of electrodeposited ternary NiMoCd alloys containg 1 at% Cd.
They claimed that the resulting low Tafel slope (26e30 mV)
obtained at elevated temperatures can be explained of the
formation of a hydride phase. Eliaz and Gileadi [21] made a
review on Mo and W alloys with transition metals. The citrate
concentration increase was reported to decrease overall cur-
rent efficiency. Citrate baths provided higher W content in
comparison with those containing tartrate or malate. The ef-
fect of temperature was proved to depend on solution
composition and increasing the current density led to higher
W content for most used electrolytes. Tasic et al. [22] studied
the electrodeposition of NieW alloys from ammoniacal-
citrate bath containing different concentrations of sodium
tungstate, on electrocatalytic activity towards HER. They
found that the films obtained at higher deposition current
densities had the lowest overvoltage for the HER and claimed
that the surface roughness of the coatings is responsible for
their electrocatalytic activity.
If the electrodeposition of NiMo and NiW binary alloys
was already approached, to the best of our knowledge, there
are very few research works on the synthesis of ternary
NiMoW alloys by electrodeposition that have been conduct-
ed. Cesiulis and co-workers [23] investigated the effect the
ratio of the Na
2
MoO
4
and Na
2
WO
4
concentrations in a pyro-
phosphate bath on the content of the ternary NieMoeW al-
loys. They reported that the deposits were crack-free for a W
content less than 5e6 at% and in all cases, the sum of Mo and
W amounts in the alloys does not exceed 15 at%. More
recently, Sun et al. [24] studied the effect of current density
on the composition of NiWMo alloys electrodeposited onto
rotating cylinder electrodes in citrate-boric acid electrolyte.
They found that (but without clearly explaining why) when
depositing NiWMo alloys in an equimolar solution, the Mo
content in the deposit is significantly higher than W content.
However, the influence of deposition parameters (electrolyte
composition, deposition potential or current density etc) on
the corrosion properties and electrocatalytic activities of
electrodeposited ternary NiMoW coatings towards the
hydrogen evolution reaction have not been investigated.
Moreover, the nucleation/growth mechanism of these
ternary alloys has never been treated. In the present work,
we report the results regarding the electrochemical aspects
of the deposition process and the mechanisms of nucleation
and growth of NiMoW alloys from slightly acidic citrate
ammonia-free electrolyte. Particular attention was paid to
the effects of both Mo/W ratio in the plating bath and
deposition potential on the corrosion resistance and
electrocatalytic activity of the alloys for the hydrogen evo-
lution reaction (HER) in concentrated alkaline media.
Experimental
The electrochemical measurements were carried out with a
Voltalab 40 potentiostat-galvanostat (Model PGZ 301)
controlled by VoltaMaster 4 software (Hach Lange GmbH,
Germany) and using a conventional three electrode cell as-
sembly at ambient (laboratory) temperature. Platinum wire
and pure copper sheets sized 1 2cm
2
were used as working
electrode, a platinum foil having a large-area to prevent po-
larization of the anode was used as counter electrode and
saturated calomel electrode (SCE) served as reference elec-
trode. To obtain reproducible results, the substrate surface
was polished with successive grades of sand paper to obtain a
mirror finish. The samples were then treated in 10% (v/v) hy-
drochloric acid (HCl) for 2 min to remove any adherent oxide
layer on the surface and rinsed with distilled water before
every experiment. The plating solutions were made from
analytical-grade chemicals (Sigma-Aldrich, USA) and doubly
distilled water and contained 0.25 M NiSO
4
$6H
2
O, 0.05 M
NiCl
2
$6H
2
O, Na
2
WO4, Na
2
MoO
4
.2H
2
O as metal sources (with
W/Mo ratio ranging from 0.5 to 2) and 0.4 M Na
3
C
6
H
5
O
7
$2H
2
O
as complexing agent. After preparation, all solutions were
deoxygenated with a stream of nitrogen within the cell before
each experiment. The plating bath pH was quasi-neutral (6.25)
adjusted by addition of potassium hydroxide or sulfuric acid
as needed and monitored with a calibrated pH-meter (WTW-
inoLab pH 7310). No agitation was utilized in all experiments.
To investigate the corrosion resistance and catalytic activ-
ities of electrodeposited NiMoW films for hydrogen evolution
reaction (HER) in a 3.5% NaCl and 30% KOH solutions respec-
tively, Tafel curves (5 mV/s scan rate from 350 mV to þ350 mV
vs OCP), cyclic voltammograms and EIS (Nyquist and Bode) plots
were performed at room temperature. The same threeelectrode
electrochemical cell used for electrodeposition study was used
for the characterization of the coatings. EIS measurements at a
given applied potential and an alternative current (AC) sine
wave of 10 mV amplitude were taken in the frequency range of
100 KHz to 10 mHz. The impedance data, such as the electrolyte
resistance Re, the charge-transfer resistance (Rct) and the
double layer capacity of the interface (Cdl) were determined
from the Nyquist and Bode plots. All the chosen specimens were
activated in 10 vol% HCl for 30 s prior to electrochemical testing.
The corrosion rate occurring at the electrochemical interface
(mm/y), corrosion potential (mV/SCE) and exchange current
density (mA/cm
2
) were calculated automatically by using Tafel
extrapolation method provided by Voltamaster 4 software
[25,26]. The structural quality of the NiMoW samples was
examined using X-ray diffraction (XRD) analysis, carried out on
a powder diffractometer (Philips X'Pert Pro Multipurpose X-ray
diffractometer) with copper Karadiation source (lKa¼1.5418
A)
in the (2q) range of 20e100. The compositional and morpho-
logical properties were investigated using a field-emission
scanning electron microscope (FESEM, JSM-7100F) with an
accelerating voltage of 10 kV and acquisition time of 90 s, and
equipped with a high resolution silicon drift detector (SDD) for
X-ray Energy Dispersive Spectroscope (EDS) microanalysis.
international journal of hydrogen energy xxx (2017) 1e12 3
Please cite this article in press as: Allam M, et al., Electrodeposition and characterization of NiMoW alloy as electrode material for
hydrogen evolution in alkaline water electrolysis, International Journal of Hydrogen Energy (2017), http://dx.doi.org/10.1016/
j.ijhydene.2017.08.012