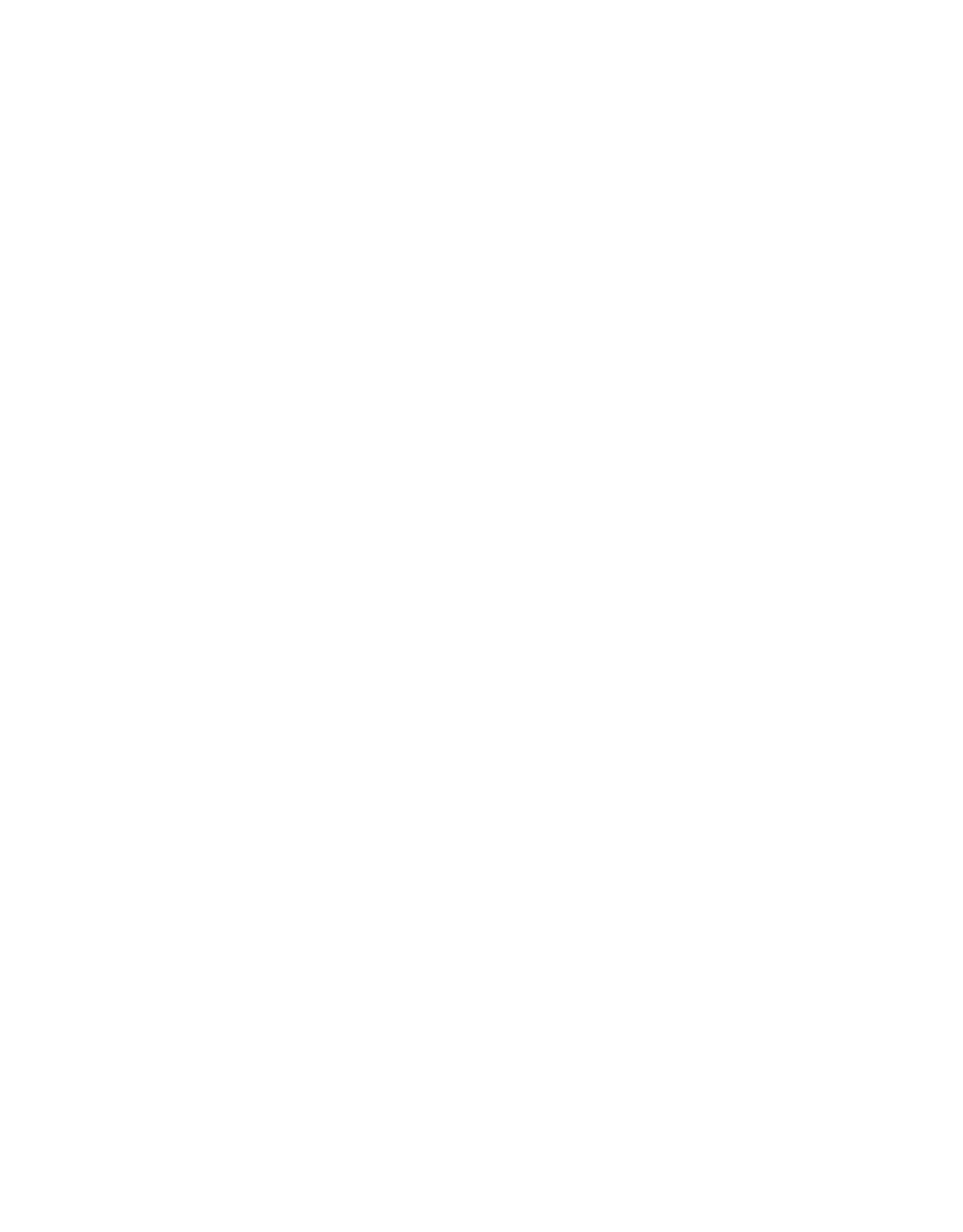
Cell, Vol. 101, 91–101, March 31, 2000, Copyright 2000 by Cell Press
Drosophila p53 Is a Structural
and Functional Homolog of the Tumor
Suppressor p53
apoptosis (Wagner et al., 1994). In fact, p21 may protect
against p53-induced apoptosis in at least some cell
types (Waldman et al., 1996; Wang and Walsh, 1996;
Gorospe et al., 1997; Bissonnette and Hunting, 1998;
Yu et al., 1998; Asada et al., 1999).
Michael Ollmann,*
§
Lynn M. Young,*
§
Charles J. Di Como,
†
Felix Karim,* Marcia Belvin,*
Stephanie Robertson,* Kellie Whittaker,*
Madelyn Demsky,* William W. Fisher,*
Andrew Buchman,* Geoffrey Duyk,* Lori Friedman,* Induction of apoptosis by p53 is critical for the tumor
Carol Prives,
†
and Casey Kopczynski*
‡
suppressor function of p53. There appear to be multiple
*Exelixis, Inc. mechanisms through which p53 promotes apoptosis.
260 Littlefield Avenue For example, p53 can transcriptionally activate the pro-
South San Francisco, California 94080 apoptotic genes Bax (Miyashita and Reed, 1995), Fas
†
Department of Biological Sciences (Owen-Schaub et al., 1995), and IGF-BP3 (Buckbinder
Columbia University et al., 1995), as well as a set of genes that may promote
New York, New York 10027 apoptosis through theformation of reactive oxygenspe-
cies (Polyak et al., 1997). Furthermore, there is evidence
that p53 can induce apoptosis in the absence of its
Summary transcriptional activation function (reviewed in Ko and
Prives, 1996).
The importance of p53 in carcinogenesis stems from The recent discovery of two p53-related genes, p63
itscentralroleininducingcellcyclearrestorapoptosis and p73, has revealed an additional level of complexity
in response to cellular stresses. We have identified a to studying p53 function in vertebrates (Kaelin, 1999).
Drosophila homolog of p53 (“Dmp53”). Like mamma- Both genes encode proteins with transactivation, DNA-
lian p53, Dmp53 binds specifically to human p53 bind- binding, and tetramerization domains, and some iso-
ing sites, and overexpression of Dmp53 induces apopto- forms of p63 and p73 are capable of transactivating
sis. Importantly, inhibition of Dmp53 function renders p53 target genes and inducing apoptosis. It was initially
cells resistant to X ray–induced apoptosis, suggesting thought that only p53 was induced in response to DNA
that Dmp53 is required for the apoptotic response to damageandotherstresssignals.Thereisnowevidence,
DNA damage. Unlike mammalian p53, Dmp53 appears however, that p73 is also activated by some forms of
unable to induce a G1 cell cycle block when overex- DNA damage in a manner that is dependent upon the
pressed, and inhibition of Dmp53 activity does not c-Abl tyrosine kinase (Agami et al., 1999; Gong et al.,
affect X ray–induced cell cycle arrest. These data re- 1999; Yuan et al., 1999). These data suggest that p53-
veal an ancestral proapoptotic function for p53 and independent apoptotic pathways may be mediated by
identify Drosophila as an ideal model system for eluci- other p53 family members.
dating the p53 apoptotic pathway(s) induced by DNA A better understanding of p53-regulated pathways
damage. could lead to improved strategies for treating p53-defi-
cient tumors. However, the apparent complexity of p53
Introduction function in vertebrates has hampered efforts to eluci-
date these pathways. The fruit fly Drosophila has proven
p53 is a tightly regulated transcription factor that in- to be a powerful tool for the genetic dissection of bio-
duces cell cycle arrest or apoptosis in response to cellu- chemical pathways. We report the identification of a
lar stresses such as DNA damage and oncogene activa- Drosophila homolog of p53 (the abbreviation “Dmp53”
tion (Ko and Prives, 1996; Levine, 1997; Giaccia and will be used here to distinguish Drosophila p53 from
Kastan, 1998; Kamijo et al., 1998). Loss or inactivation other family members). Dmp53 shares significant amino
of the p53 tumor suppressor gene is the single most acid identity with the DNA-binding domain of p53, in-
commonmutationinhumancancer(Hainautetal.,1998). cluding conservation of key residues commonly mu-
Thus, inactivation of p53 function may provide a selec- tated in human cancer. Like p53, overexpression of
tiveadvantagetotumorcells throughaccruedmutations Dmp53 induces apoptosis, and inhibition of Dmp53
leading to deregulated cell proliferation and resistance functionrenderscellsresistanttoXray–inducedapopto-
tocelldeath(Graeberetal.,1996;Kinzler andVogelstein, sis. In contrast to mammalian p53,Dmp53 overexpres-
1996). sion does not induce aG1 cell cycle block, andinhibition
Numerousstudies haveestablishedthat growtharrest of Dmp53 activity does not affect X ray–induced cell
and apoptosis are independent functions of p53 (re- cycle arrest. These data suggest that the ancestral func-
tion of p53 may have been restricted to eliminating dam-
viewed in Gottlieb and Oren, 1996). p53-dependent G1 aged cells by apoptosis and identify Drosophila as an
arrest occurs largely through transcriptional induction ideal model system for dissecting p53-mediated apo-
of p21
WAF1
, which prevents entry into S phase by inhib- ptotic pathways.
iting G1 cyclin–dependent kinase activity (el-Deiry et al.,
1993; Harper et al., 1993; Xiong et al., 1993; Deng et al., Results
1995). However, p21 is not required for p53-dependent
Identification of Dmp53
‡
To whom correspondence should be addressed (e-mail: caseyk@
We identified a partial Drosophila cDNA with sequence
exelixis.com).
§
These authors contributed equally to this work.
similarity to the p53 family of genes as a result of a