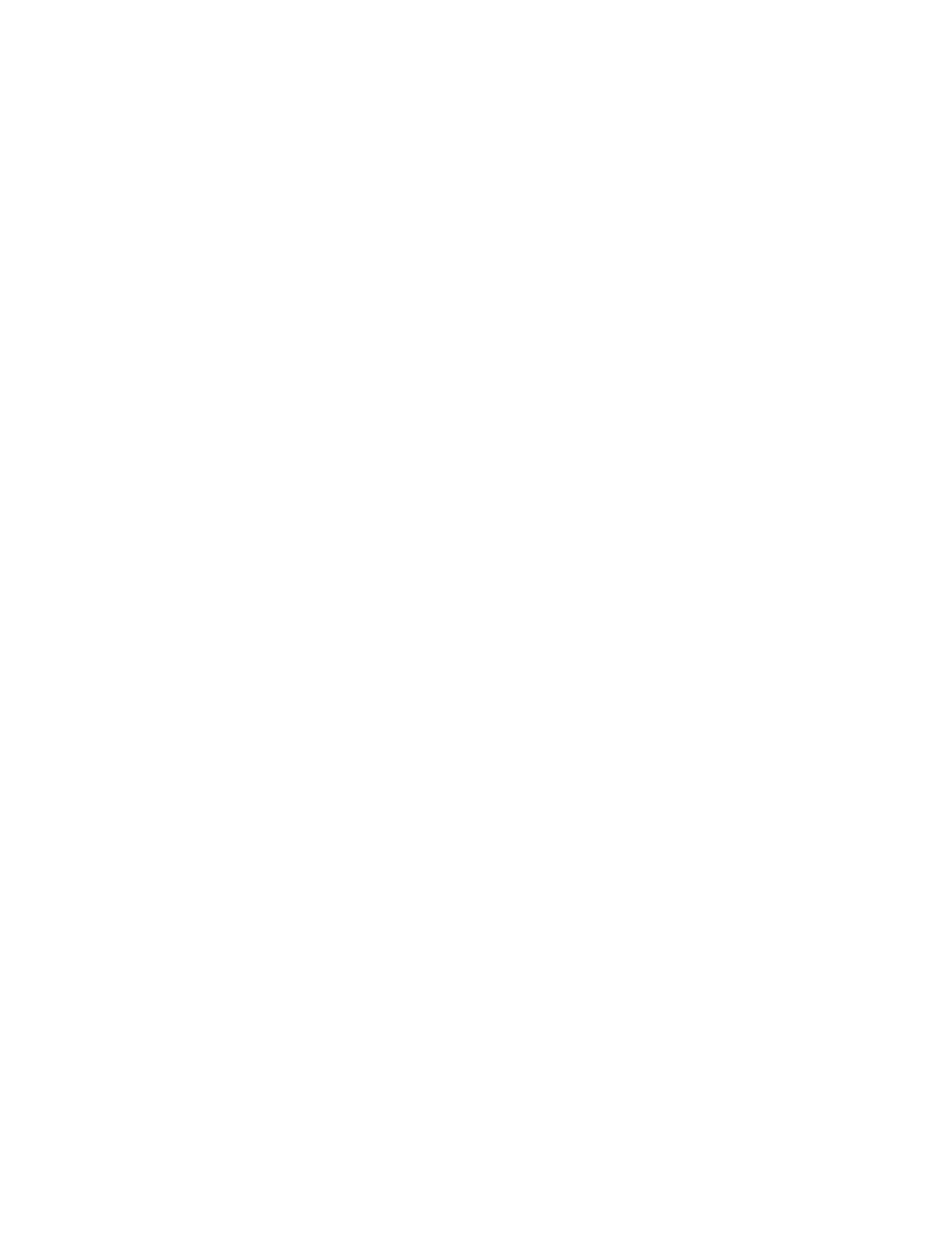
could readily occur (Ho et al., 1997a; Kuwabara et al.,
2003).
Formes Frustes of GBS Also Exist as
Regional Variants
The regional variants of GBS only paralyse specific
areas of the body, such as the eyes or face, or the
afferent sensory and autonomic systems (Ropper,
1994). The most widely studied of these variants is the
MFS (Fisher, 1956; Willison and O’Hanlon, 1999).Our
understanding of MFS was revolutionised following
Chiba and Kusunoki’s discovery of the anti-GQ1b anti-
body marker (Chiba et al., 1992; 1993). Since the syn-
drome was first described in 1956 as the discrete clinical
triad of ophthalmoplegia, ataxia, and areflexia, anti-GQ1b
antibody testing has allowed MFS to evolve nosologi-
cally and now encompasses closely related formes
frustes, mainly characterised by acute cranial motor neu-
ropathies with ataxia – the anti-GQ1b antibody syn-
dromes (Odaka et al., 2001; Paparounas, 2004).Inthe
Bickerstaff’s encephalitis, MFS-like features occur in
conjunction with brain stem involvement, comprising
pyramidal tract signs and impaired consciousness, and
two thirds of cases are anti-GQ1b antibody positive
(Bickerstaff and Cloake, 1951; Odaka et al., 2003).The
close relationship between GBS and MFS is considered
because it should direct our search towards common
underlying immunopathological mechanisms. Thus,
some MFS cases merge into confluent GBS with
respiratory and limb involvement, and similarly, some
GBS cases also evolve to develop an MFS pattern of
clinical involvement – in these overlapping cases, anti-
GQ1b antibodies are generally detected. MFS variants
include solitary ophthalmoplegia or ataxia and orophar-
yngeal weakness without ophthalmoplegia (O’Leary
et al., 1996). Viewed in its broadest contemporary
sense, MFS could be considered as a PNS/CNS overlap
syndrome with extremely variable degrees of involve-
ment of particular central or peripheral anatomical sites
in individual cases, with all permutations being highly
associated with anti-GQ1b and anti-GT1a antibodies
(O’Leary et al., 1996; Odaka et al., 2001).
The selective affliction of cranial and in particular
extraocular nerves in the anti-GQ1b antibody syn-
dromes is believed to be due to the enrichment of
the target antigen(s) in affected sites (Chiba et al.,
1997). However, there are clearly additional levels of
complexity influencing the clinical phenotype that
remain undiscovered. What is notable by its absence
is involvement of extraocular motor nerves in AIDP,
arguing that the AIDP antigen(s) may analogously be
relatively enriched in limb and axial myelin (in compar-
ison with extraocular motor nerve myelin) as opposed
to being a generic myelin antigen(s). This might
mitigate against the major structural proteins of myelin
being dominant AIDP antigens. A chronic ataxic neuro-
pathy has also been reported that may be associated
with relapsing ophthalmoplegia in some cases; such
patients often have anti-ganglioside antibodies, includ-
ing anti-GQ1b, which are persistently present, usually
occurring as IgM paraproteins (Willison et al., 2001).
Anti-Nerve Antibodies in GBS: Lessons
From Rodent Models
Great progress has been made in correlating clinical
phenotypes with serological profiling of anti-nerve antibo-
dies, covering a wide spectrum of peripheral nerve anti-
gens. The myelin protein-specific T and B cell-mediated
rodent model of GBS (experimental allergic neuritis [EAN])
was described 50 years ago and has yielded many impor-
tant experimental insights into peripheral nerve inflamma-
tion (Spies et al., 1995; Rostami, 1997; Gabriel et al., 1998;
Taylor and Pollard, 2003). However, translational studies
identifying equivalent immune responses in human neuro-
pathy cases have been more modest (Gabriel et al., 2000;
Ritz et al., 2000; Yan et al., 2000; Favereaux et al., 2003;
Latov and Renaud, 2004). Whether further research will
overcome this remains to be seen.
In contrast, the progress in identifying myelin and
axonal glycolipids as antigens has been somewhat
more forthcoming. Interestly that ganglioside-/glycolipid-
induced rabbit EAN was also first described several
decades ago (Nagai et al., 1976; Saida et al., 1979),
but in contrast to myelin protein-induced EAN, was
then virtually neglected for approximately 20 years,
and has now been revived with compelling data that
reinforce many recent clinical findings. Thus, a series
of recent rabbit immunisation studies have led to the
generation of models of anti-GD1b antibody-associated
ataxic neuropathy and anti-GM1 antibody-associated
motor axonal neuropathy that mimic many of the clin-
ical and pathological features of the human syndromes
(Kusunoki et al., 1996; Yuki et al., 2001; 2004).
These studies have provided some of the most
compelling evidence that anti-ganglioside antibodies
and their effector pathways can induce clinically rele-
vant phenotypes. In this respect, ganglioside-induced
EAN has come full circle. The return of researchers,
principally in Japan, to these early rabbit studies was
driven in large part by the clinical identification of anti-
ganglioside antibodies in GBS cases, starting in 1988
(Ilyas et al., 1988) and escalating in scope ever since. A
wealth of clinical serological associations have now been
described and the topic reviewed regularly (Yuki, 2001;
Willison and Yuki, 2002). In summary, in human disease,
anti-GM1, anti-GD1a, anti-GM1b, and anti-GalNAcGD1a
antibodies are highly associated with AMAN (Ogawara
et al., 2000) and anti-GQ1b, anti-GT1a, anti-GD3, and
Willison Journal of the Peripheral Nervous System 10:94–112 (2005)
96