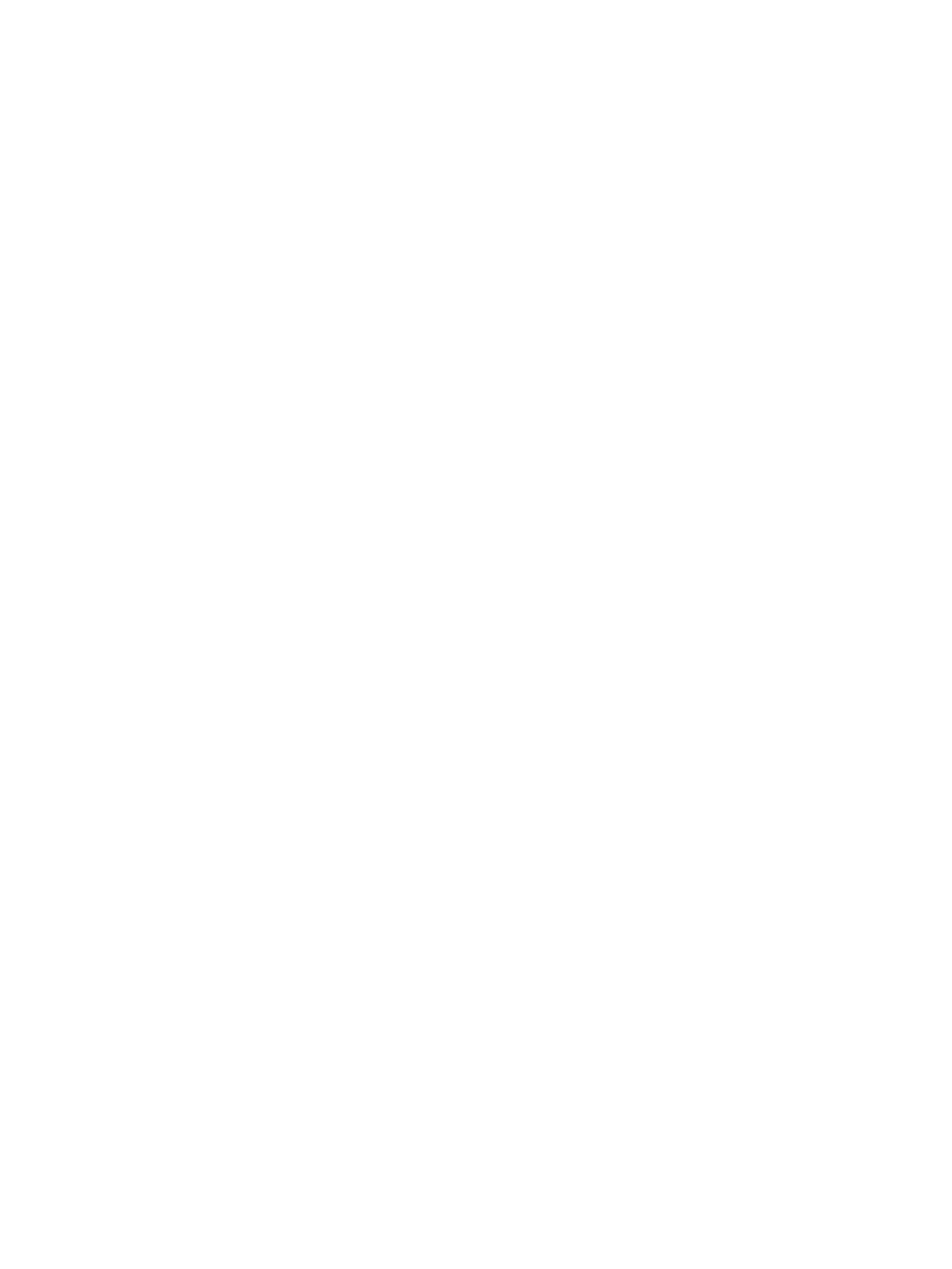
sensitivity of species recognition, especially in groups that
have undergone recent or cryptic speciation (Padial & De
La Riva, 2009).
The identification of cryptic species is critically impor-
tant for many reasons, including accurate assessment of
biodiversity estimates, facilitating disease and crop plant
pathogen control, and directing conservation efforts
towards vulnerable endemic species (Bickford et al.,
2007). In addition, cryptic speciation may result from
recently evolved sibling species where species limits can
be ambiguous because morphological differences have
not yet accumulated (Knowlton, 1993). These recently
evolved species are good candidates for speciation
research because these groups more closely meet the
assumption that the species’ geographic range has not
changed over time (Losos & Glor, 2003). Further,
research into cryptic species limits can help to both
identify and conserve the processes currently driving
speciation in various groups and regions (Carnaval et al.,
2009).
Situated off the south-eastern coast of Africa, the
island of Madagascar is a model region for cryptic species
identification and studies of speciation (Vences et al.,
2010). Madagascar and Greater India first broke away
from Africa as early as 165 Ma, and Madagascar has
been isolated since separating from Greater India about
88 Ma (Storey et al., 1995). This island continent has a
complicated and poorly understood paleoclimatic his-
tory, but some information is known. Madagascar
experienced a generally dry environment when first
separated from India (Wells, 2003), but the overall
climate became more humid as it drifted northwards
towards the equator. However, the climate again became
drier and cooler during glacial periods, with areas at
lower elevations experiencing more pronounced aridifi-
cation than those at higher elevations (Haffer, 1969).
Presently, the island is composed of striking environ-
mental heterogeneity, with habitat transitions occurring
abruptly. Trade winds and orographic uplift ensure
regular rainfall on the north-east and eastern coast,
and the central mountain chain acts as a barrier causing
a rainfall gradient from the humid north-eastern and
eastern rainforests to the south-western spiny deserts
(Jury, 2003). In addition, there is complex topography
(maximum elevation 2876 m), seasonal rainfall patterns
and drainage systems, all which contribute to the
environmental complexity.
Several hypotheses about the mechanisms driving spe-
ciation in Madagascar have been proposed, and many of
these are related to either the paleoclimate or current
climate of the island. The Watershed hypothesis (Wilme
et al., 2006) proposes that glaciation periods resulting in
severe arid conditions at lower elevations caused species to
move to higher elevations towards more humid areas.
Species distributed in lower elevation watersheds
became trapped in arid pockets, and adapted and diversi-
fied in isolation. The Montane Endemism hypothesis
(Raxworthy & Nussbaum, 1995; Wollenberg et al., 2008)
proposes that some populations of species broadly distrib-
uted during glacial periods became isolated on mountain-
tops during warmer interglacials. The hypothesis of
ecologically mediated speciation (Raxworthy et al., 2007,
2008) proposes that the niches of sister species become
divergent as they adapt to ecotones under disruptive
selection and assortative mating. The Riverine Boundary
hypothesis (Pastorini et al., 2003; Goodman & Ganzhorn,
2004) proposes that the river systems in Madagascar have
restricted gene flow causing isolation between popula-
tions. Lastly, the Ecogeographic Constraint hypothesis
(Yoder & Heckman, 2006) proposes that the abrupt
distinction between the climates, rainfall patterns, and
vegetation of eastern and western Madagascar allows for
initial east–west divergence within widely distributed
species, with subsequent speciation constrained within
eastern and western regions. This hypothesis is an
example of ecologically mediated speciation, where initial
speciation can be either allopatric or parapatric.
Understanding the processes driving speciation in
Madagascar is of interest because an exceptional number
of new endemic species continue to be described (Myers
et al., 2000; Vieites et al., 2009). Some of these new
descriptions have resulted from the splitting of previously
recognized monotypic genera. For example, until
recently (see Miller, 1977) mouse lemurs (Microcebus)
were considered to be a single species (Microcebus muri-
nus), but are now split into at least 15 species using
phylogeographic and morphometric analyses (Rasoloari-
son et al., 2000; Yoder et al., 2000; Louis et al., 2006,
2008). Many other phylogeographic studies have shown
other taxa in Madagascar to represent complexes of
cryptic species (e.g. Raxworthy et al., 2007; Raselima-
nana et al., 2009). In particular, the identification of
recently evolved species, which are often cryptic, offers
important opportunities for improving our understand-
ing of the processes that drive speciation in Madagascar.
A candidate cryptic species complex is the carpet
chameleon Furcifer lateralis (Gray, 1845), which is
endemic to Madagascar. The carpet chameleon is a
popular and familiar species in the pet trade and
consequently is exported in high numbers. Less known
is that F. lateralis is also an excellent model organism for
understanding the processes driving speciation. Although
most Malagasy chameleons exhibit considerable regional
endemism, paradoxically, F. lateralis is one of the most
widely distributed endemic reptiles in Madagascar (Rax-
worthy et al., 2003). The species is distributed in nearly
all areas of Madagascar, with the exception of montane
regions above 1780-m in elevation and a small high
precipitation region in the north-east. It is also often
found at high population densities in severely degraded
forests, grasslands, agricultural areas and urban environ-
ments (Karsten et al., 2009; Randrianantoandro et al.,
2009). This chameleon exhibits subtle regional variation
in axillary pit development, white line development
1400 A. M. FLORIO ET AL.
ª2012 THE AUTHORS. J. EVOL. BIOL. 25 (2012) 1399–1414
JOURNAL OF EVOLUTIONARY BIOLOGY ª2012 EUROPEAN SOCIETY FOR EVOLUTIONARY BIOLOGY