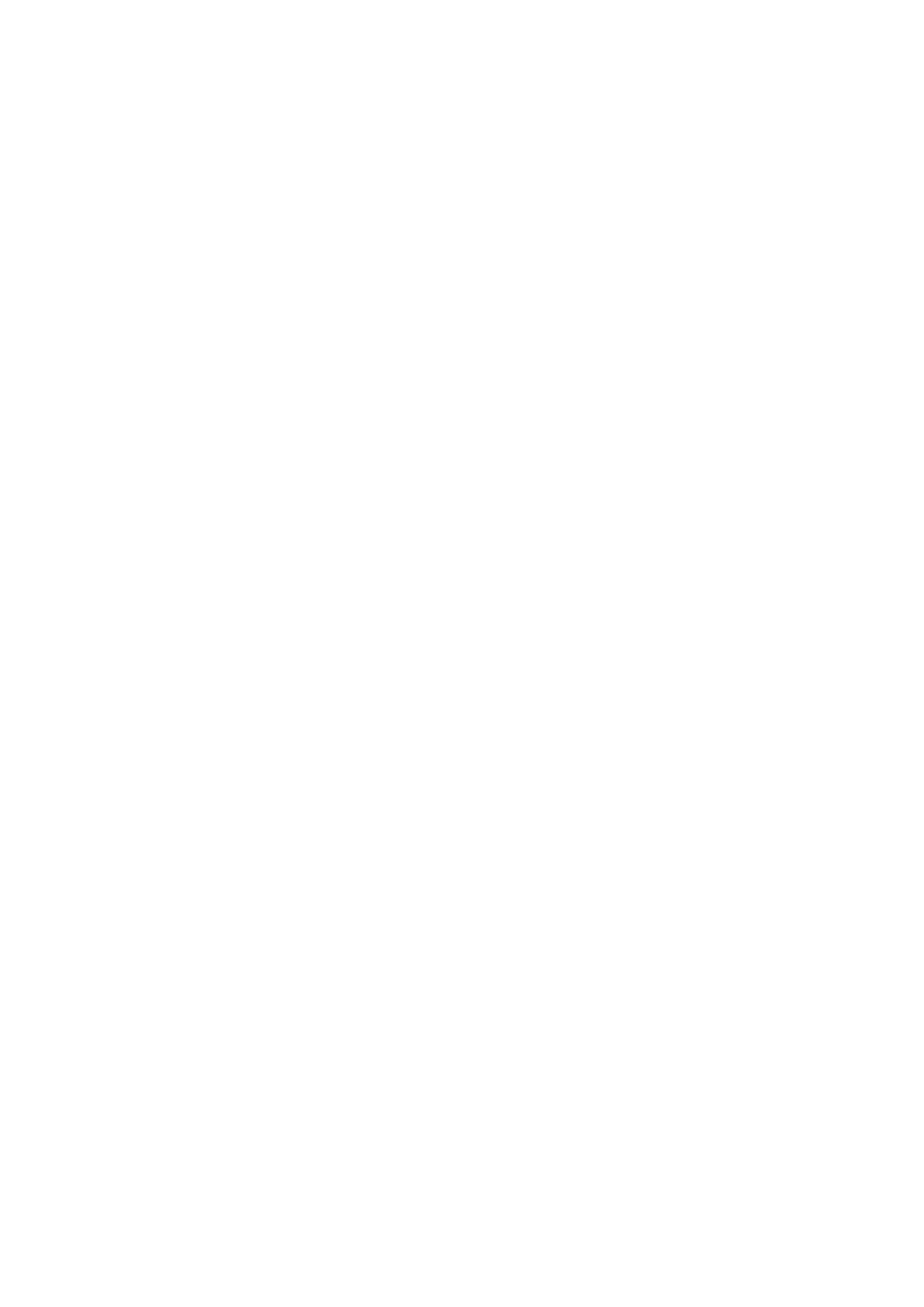
Remerciements
Je tiens tout d’abord à remercier Beat Oertli pour m’avoir fait découvrir le monde des mares et
étangs et pour m’avoir donné l’opportunité de me lancer dans cette grande aventure. Un grand
merci pour avoir passé du temps à relire et encore relire mes articles et pour m’avoir poussée à me
dépasser.
Je remercie également Anthony Lehmann de m’avoir initiée à GRASP et d’avoir toujours été très
disponible pour répondre à mes questions statistiques ainsi que de m’avoir accueillie à Battelle pour
finir cette thèse.
Je remercie également Dominique Vallod pour m’avoir fait découvrir les étangs de la Dombes
scientifiquement lors de discussions sur leur fonctionnement ou concrètement en participant à une
pêche ainsi que pour sa grande disponibilité.
Je tiens également à remercier Michael Samways (Université de Stellenbosch) et Emmanuel Castella
(Université de Genève) d’avoir accepté d’évaluer le présent travail.
Ce travail n’aurait pas pu aboutir sans l’aide de nombreuses personnes qui ont participé aux
campagnes de terrain, au travail de laboratoire et à la gestion des bases de données. Je remercie
toute l’équipe PLOCH du LEBA, toute l’équipe d’hepia, de l’ISARA et de l’Université de Lyon. Je
remercie vivement Pascale Nicolet et Jane Park qui ont toutes les deux corrigés avec brio l’anglais de
mes publications.
Ce travail a également bénéficié de ma collaboration développée en Afrique du Sud avec le
Département d’Ecologie de la Conservation et d’Entomologie. Je remercie vivement Michael
Samways pour m’avoir si bien accueillie et pour les discussions constructives et John Simaika pour
tous les moments partagés ainsi que toutes nos discussions animées.
Je remercie les différentes institutions, fondations et collectivités qui ont participé au financement de
ce travail : l’Office fédéral suisse de l’environnement (OFEV), de nombreux cantons suisses,
l’Université de Genève (Laboratoire d’Ecologie et de Biologie Aquatique), la HES-SO (RCSO RealTech),
la commission de recherche du Parc National Suisse, l’Agence de l’Eau Rhône Méditerranée Corse, le
Département français de l’écologie, de l’énergie, du développement durable et de la mer, la PEP
Aquaculture Rhône-Alpes et la fondation Vérots. Ce travail a également bénéficié de la participation
des propriétaires d’étangs dombistes ayant participé à l’étude. Je remercie également pour leur
soutien financier lors de congrès et de déplacements en Afrique du Sud : le Programme de recherche
commune entre Suisse et Afrique du Sud, les relations internationales HES-SO, la Société Suisse