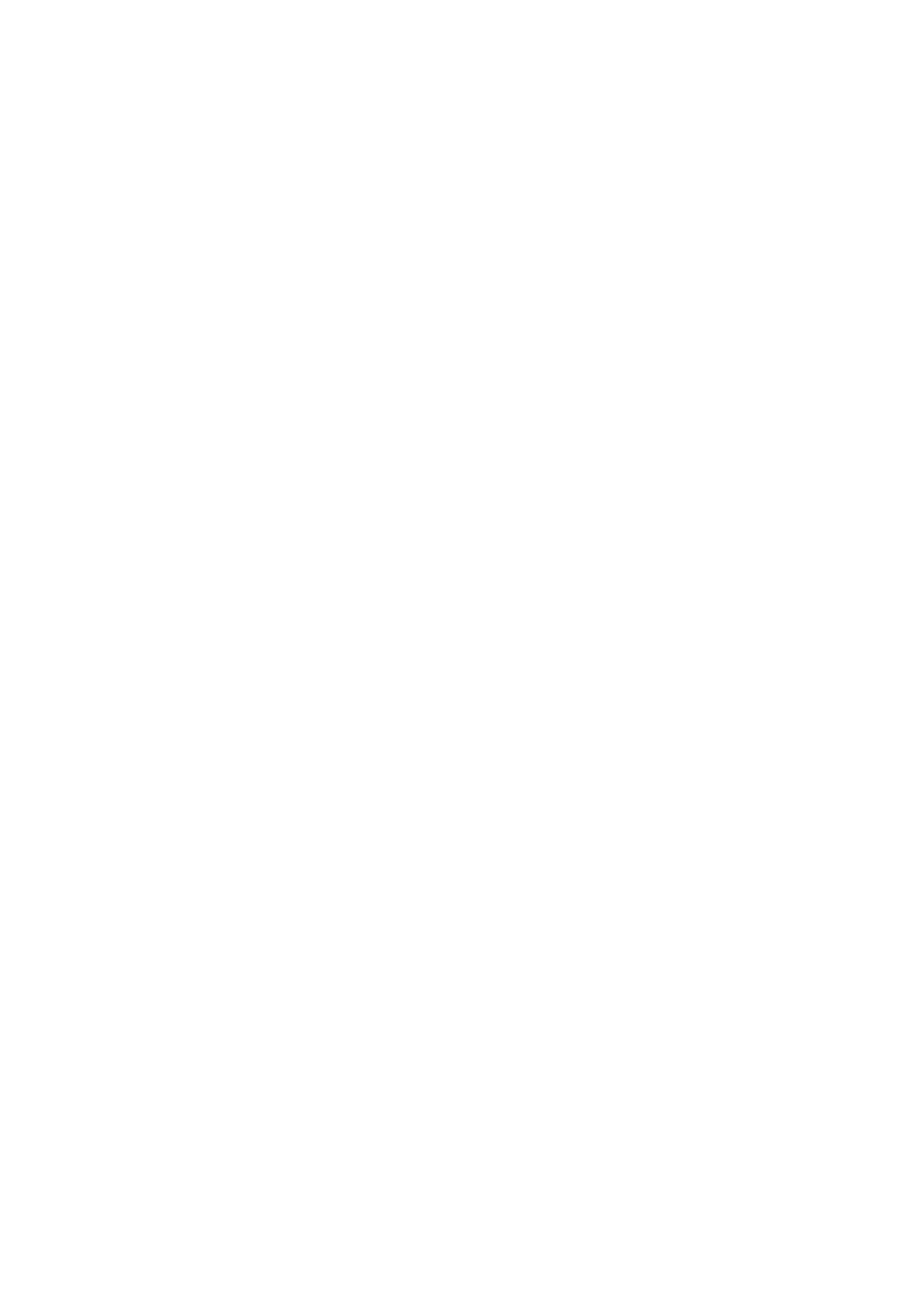
2
Acknowledgment
First and foremost, I would like to express the deepest appreciation to my “science parents”: Prof.
Catherine Muller and Prof. Philippe Valet for inspiring me and for providing such an interesting
project for me to work during these four years. Thank you for your direction and your help in the
development of my scientific mind: innovative concept, insistence, enthusiasm, strict for the
science, and how to give a speech in front of people. I am honored to have worked with you and I
have learned more from you than you will know.
Thanks to the past members in MIKA team: thanks to Bea not only for teaching me many
experiment skills from my very beginning and start this interesting project, but also teaching me
how to make crêpe and Moelleux a l`ananas et noix de coco, and also all the “useful French words”
. I am really happy to have you such a kind and straight “little boss” and French friend. Thanks to
Lud for picking me at the train station when I first arrived in Toulouse and helping me a lot from
the life to the work at the beginning. Thanks to Cathy Botanch for your welcome cake and your
kindness.
Thanks to the present members in our team, you are really helpful and friendly: Steph for teaching
me how to use confocal microscopy and all the IF analysis; Laurence, for reviewing my thesis
manuscript in spite of your very busy schedule and I am enjoying discussing with you; The
“Prostate boys”: Victor and Adrien, the rest “Breast girls” Camille Lehood, hey chouette! and
Delphine, “Melanoma princess” Ikrame and “Hypoxia queen” Fred, Your lively discussion at group
meeting have made science come to life, I have truly valued our scientific interaction. I treasure all
the fun we have made during these years and each time we got together, running, eating and
drinking… and you are also very good French teacher “Ca roule ma poule” “c’est parti mon kiki” I
will frequently repeat them in order to keep link with you
Thanks to “Adipolab” members: especially, “Mice queen” Sophie for helping and teaching me the
in vivo experiment, Camille for the β-oxidation detection and Cedric for providing me several
antibodies.
Thanks to Madama Escourrou Ghislaine for all Immunohistochemistry analysis and I learnt a lot
about “the microcosmic world” of breast cancer.
Thanks to other person in IPBS: Leyre, Guillaume, Piere, Emma, Ying, Jin, Zhong, Jiahui for you
kind help.
Thanks to my thesis committee members: for your encouragement, insightful comments and hard
questions.
I would like to acknowledge the financial support from CSC for 4 years that made my Ph.D work
possible.
I have been fortunate to come across many funny and good Chinese friends: SuSu, Liang, Tian,
Xiaoqian, Jian, Hang, Han, Lijun,Mingchun et al. without you, life would be bleak. Thanks for the
movies, dinners, sports, concerts and plays we enjoy together.
My sincere thanks also go to Prof. Ren GuoSheng, for offering me this opportunity to study abroad.
Lastly, I am profoundly thankful for the love, support and understanding from my parents, my
grandma!
Thank Toulouse! This sunny, lovely and peaceful city! Thank all of you!