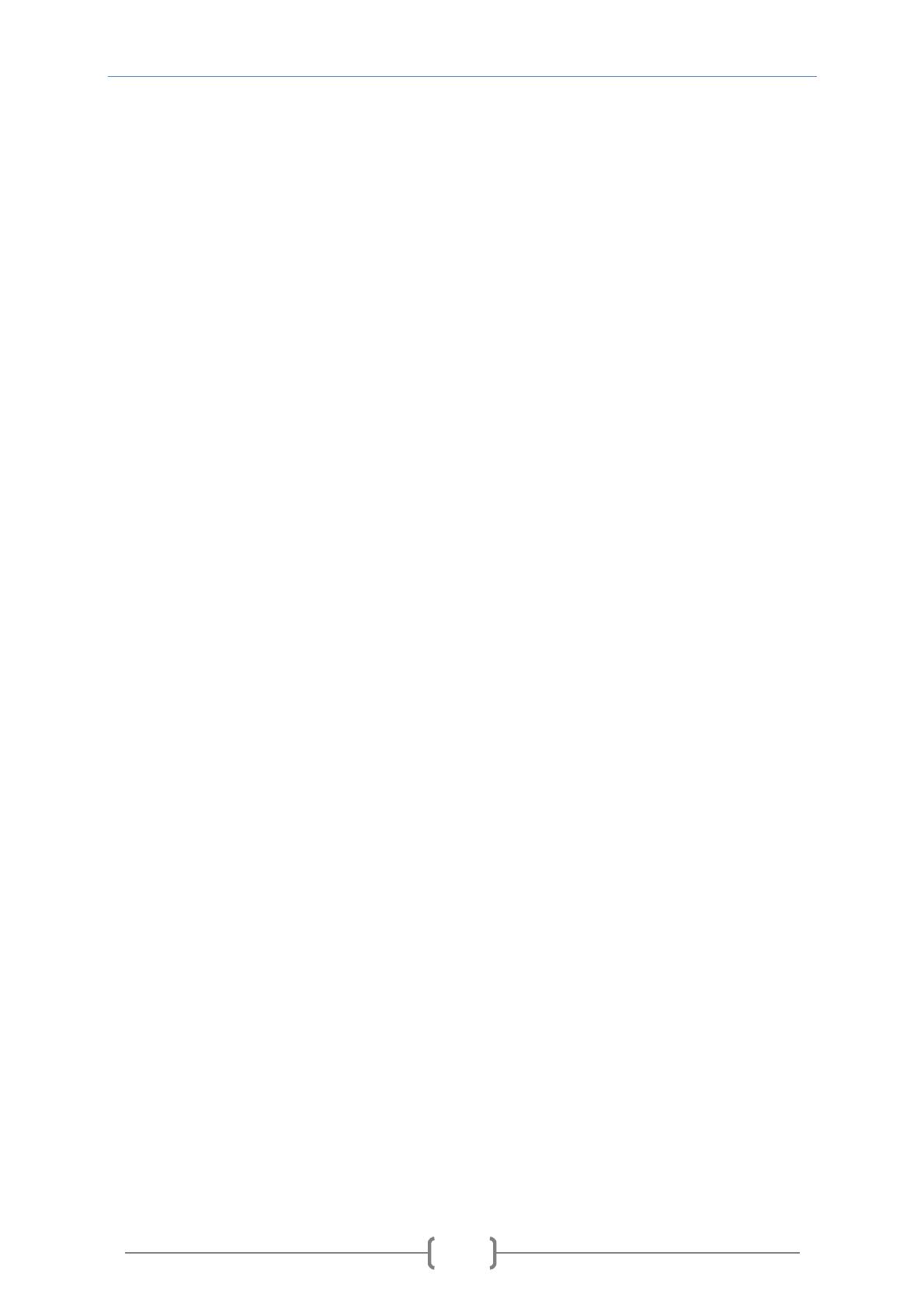
TABLE DES MATIERES
2
But du travail .................................................................................................... 46
III. RESULTATS .................................................................................................. 48
1. Les désacétylases d’histones : cibles thérapeutiques dans le cancer
canalaire pancréatique ..................................................................................... 49
1.1. Le SAHA réduit in vitro la croissance cellulaire de la lignée BxPC-3 ..................................... 49
1.2. L’inhibition spécifique d’HDAC1 ou 3 diminue in vitro la croissance cellulaire de la lignée
BxPC-3 .......................................................................................................................................... 50
1.3. Le MS-275 induit in vitro, une accumulation de la protéine et du transcrit de COX-2 dans la
lignée BxPC-3 ................................................................................................................................ 52
1.4. Implication du facteur de transcription NF-ĸB dans l’accumulation de COX-2 induite par le
MS-275 ......................................................................................................................................... 56
1.4.1. Le MS-275 induit in vitro la transcription du gène codant pour l’Interleukine 8 (IL-8) .. 56
1.4.2. Le MS-275 induit l’accumulation de la sous-unité p65 sur la chromatine...................... 57
1.4.3. L’inhibition de l’activation de NF-ĸB diminue l’expression de COX-2 induite par le
MS-275 ..................................................................................................................................... 58
1.4.4. L’inhibition spécifique par siRNA de la traduction de p65 bloque l’accumulation de la
protéine COX-2 induite par le MS-275 ...................................................................................... 59
1.5. Intérêt de l’inhibition concomitante des HDAC1/3 et de COX-2. ......................................... 60
1.6. Estimation de l’IC50 du Celecoxib sur la croissance cellulaire ............................................. 60
1.7. La combinaison MS-275/Celecoxib bloque la croissance cellulaire de la lignée BxPC-3 ....... 61
1.8. La combinaison MS-275/Celecoxib n’induit pas l’apoptose de la lignée BxPC-3 .................. 62
1.9. La combinaison MS-275/Celecoxib bloque le cycle cellulaire en phase G0/G1 .................... 63
2. Validation du concept de co-inhibition sur d’autres lignées cancéreuses
pancréatiques .................................................................................................. 67
3. Le modèle de croissance tumorale basé sur la membrane chorioallantoïque
d’embryon de poule ......................................................................................... 70
3.1. Caractérisation des tumeurs BxPC-3 développées sur CAM ................................................ 71
3.1.1. Analyse morphologique de tumeurs pancréatiques développées sur CAM............... 71
3.1.2. Caractérisation de la vascularisation intra tumorale ................................................. 73
3.1.3. Analyse descriptive et comparaison succincte à la pathologie humaine ................... 74
3.1.4. Expression de biomarqueurs du PDAC par les tumeurs développées sur CAM ......... 76
4. Validation du concept de co-inhibition sur des tumeurs développées sur
CAM ................................................................................................................. 77
IV. DISCUSSION ................................................................................................ 80