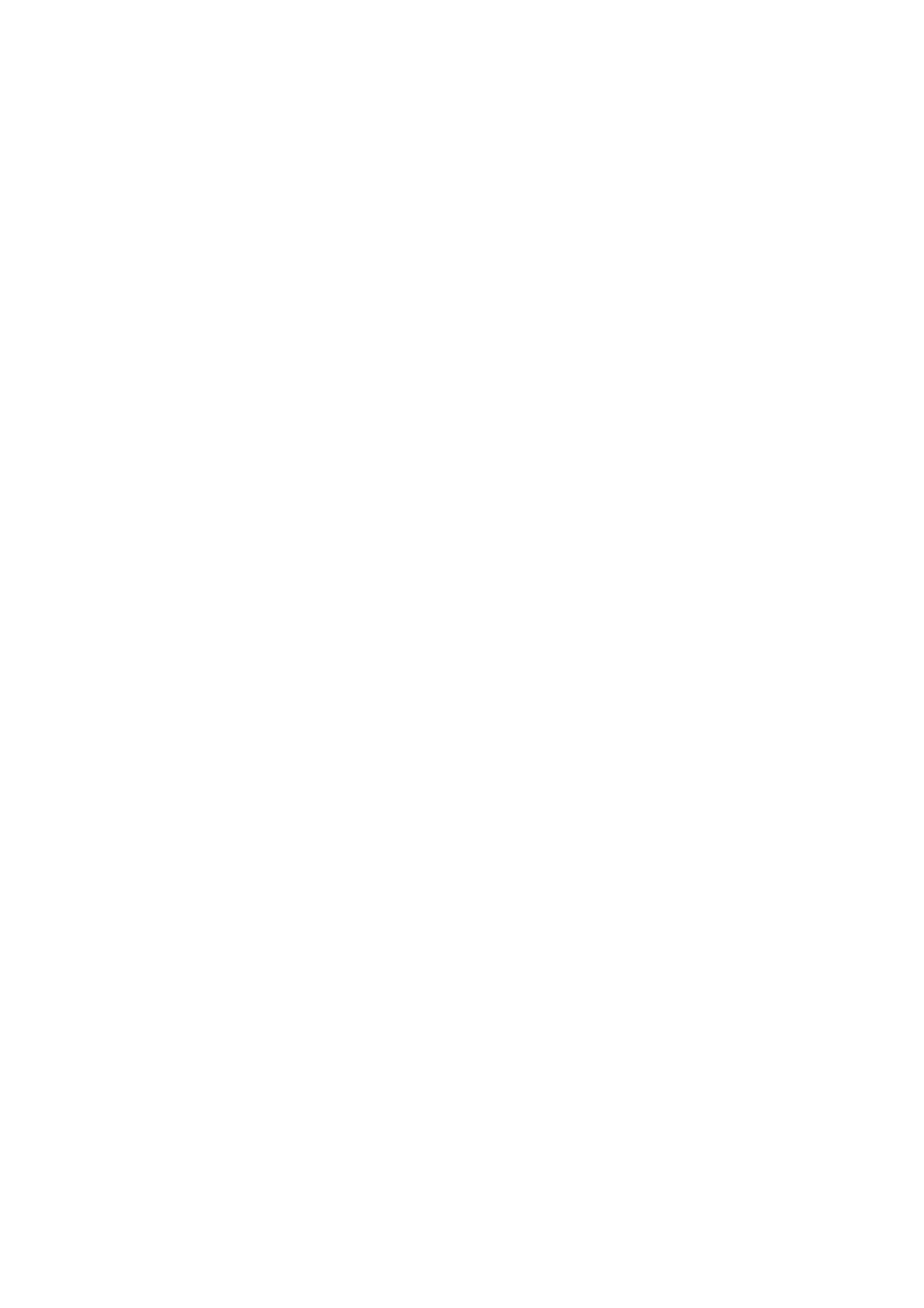
II
Agradecimento especial
Às minhas orientadoras, Regui e Fá, por terem me conduzido
no caminho da verdadeira ciência através de exemplos de
seriedade, honestidade, dedicação e objetividade. Por todas
as oportunidades que me proporcionaram, pela confiança,
atenção, orientação dedicada e presença constante, que foram
fundamentais para o meu crescimento pessoal e para a
elaboração deste trabalho, meus sinceros agradecimentos.