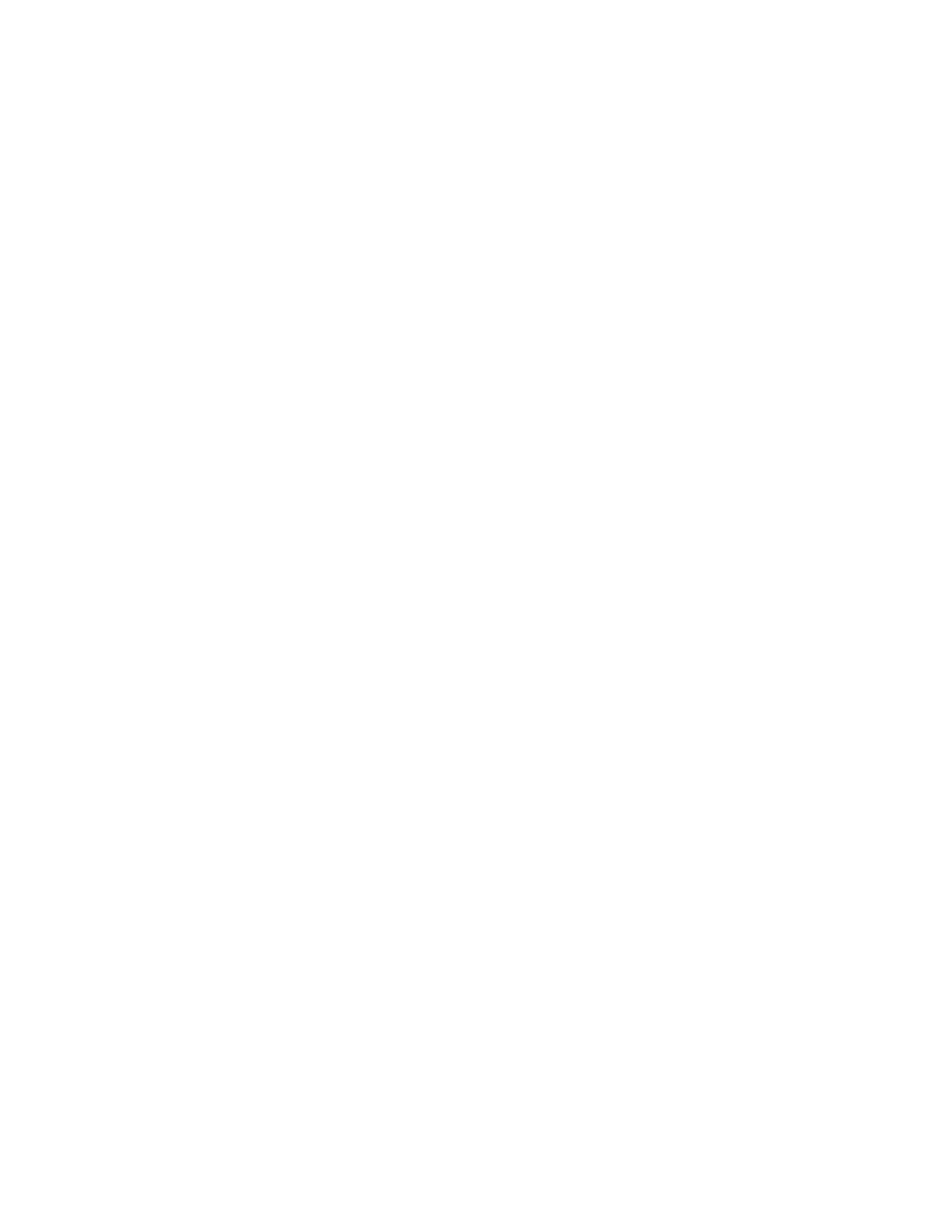
IV
Résumé
La phagocytose est un processus cellulaire par lequel de larges particules sont
internalisées dans une vésicule, le phagosome. Lorsque formé, le phagosome
acquiert ses propriétés fonctionnelles à travers un processus complexe de maturation
nommé la biogénèse du phagolysosome. Cette voie implique une série d’interactions
rapides avec les organelles de l’appareil endocytaire permettant la transformation
graduelle du phagosome nouvellement formé en phagolysosome à partir duquel la
dégradation protéolytique s’effectue. Chez l’amibe Dictyostelium discoideum, la
phagocytose est employée pour ingérer les bactéries de son environnement afin de se
nourrir alors que les organismes multicellulaires utilisent la phagocytose dans un but
immunitaire, où des cellules spécialisées nommées phagocytes internalisent, tuent et
dégradent les pathogènes envahissant de l’organisme et constitue la base de
l’immunité innée. Chez les vertébrés à mâchoire cependant, la transformation des
mécanismes moléculaires du phagosome en une organelle perfectionnée pour
l’apprêtement et la présentation de peptides antigéniques place cette organelle au
centre de l’immunité innée et de l’immunité acquise. Malgré le rôle crucial auquel
participe cette organelle dans la réponse immunitaire, il existe peu de détails sur la
composition protéique et l’organisation fonctionnelles du phagosome. Afin
d’approfondir notre compréhension des divers aspects qui relient l’immunité innée et
l’immunité acquise, il devient essentiel d’élargir nos connaissances sur les fonctions
moléculaire qui sont recrutées au phagosome.
Le profilage par protéomique à haut débit de phagosomes isolés fut
extrêmement utile dans la détermination de la composition moléculaire de cette
organelle. Des études provenant de notre laboratoire ont révélé les premières listes
protéiques identifiées à partir de phagosomes murins sans toutefois déterminer le ou
les rôle(s) de ces protéines lors du processus de la phagocytose (Brunet et al, 2003;
Garin et al, 2001). Au cours de la première étude de cette thèse (Stuart et al, 2007),
nous avons entrepris la caractérisation fonctionnelle du protéome entier du
phagosome de la drosophile en combinant diverses techniques d’analyses à haut débit
(protéomique, réseaux d’intéractions protéique et ARN interférent). En utilisant cette