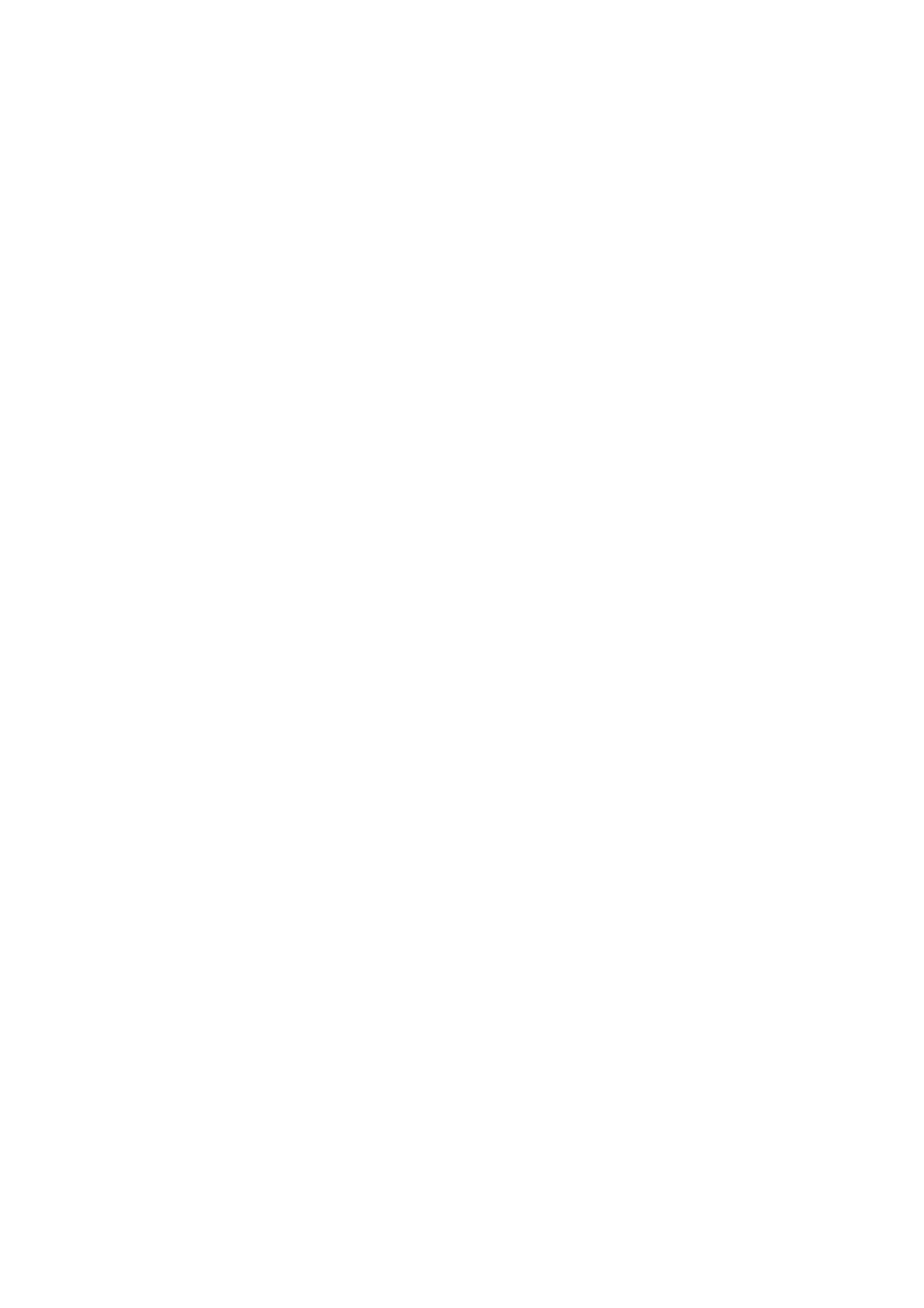
IV. IMPORTANCE CLINIQUE DE L’INTERACTION CARDIO-PULMONAIRE SUR LE DÉBIT
CARDIAQUE SYSTÉMIQUE.........................................................................................................25
V. EFFETS DE LA VENTILATION MÉCANIQUE SUR LES CONDITIONS DE CHARGE ET LES
ÉJECTIONS VENTRICULAIRES...................................................................................................26
C. MONITORAGE HEMODYNAMIQUE DES DEFAILLANCES
CIRCULATOIRES : REVUE DE LA LITTERATURE....................................................28
I. TECHNIQUES NON INVASIVES MONITORANT QUALITATIVEMENT LES DEFAILLANCES
CIRCULATOIRES .......................................................................................................................30
1. LE MONITORAGE CLASSIQUE : FREQUENCE CARDIAQUE, PRESSION ARTERIELLE NON INVASIVE
ET PLETHYSMOGRAPHIE ..........................................................................................................30
2. LA CAPNOGRAPHIE EXPIREE.............................................................................................32
3. MESURE DE LA PRESSION TRANSCUTANEE EN CO2 (TCPCO2).............................................32
II. TECHNIQUES NON INVASIVES MONITORANT QUANTITATIVEMENT LES DEFAILLANCES
CIRCULATOIRES .......................................................................................................................33
1. TECHNIQUES NON INVASIVES MONITORANT LA REPONSE AU REMPLISSAGE VASCULAIRE CHEZ
LES PATIENTS VENTILES MECANIQUEMENT...............................................................................33
2. TECHNIQUES NON INVASIVES MONITORANT LA REPONSE AU REMPLISSAGE VASCULAIRE CHEZ
LES PATIENTS EN VENTILATION SPONTANEE: LE LEVER DE JAMBES PASSIF ..................................33
3. TECHNIQUES NON INVASIVES MONITORANT LA MESURE DE DEBIT CARDIAQUE CHEZ LES
PATIENTS DES SOINS INTENSIFS................................................................................................34
a. Mesure du débit cardiaque moyennant la méthode Fick appliquée au CO2. ...........34
b. Mesure du débit cardiaque moyennant la méthode d’impédancemetrie thoracique.35
c. Mesure du débit cardiaque moyennant la méthode du Doppler oesophagien..........36
d. Mesure du débit cardiaque moyennant l’échocardiographie-Doppler.....................39